Thank you for visiting nature.com. You are using a browser version with limited support for CSS. To obtain the best experience, we recommend you use a more up to date browser (or turn off compatibility mode in Internet Explorer). In the meantime, to ensure continued support, we are displaying the site without styles and JavaScript.
- View all journals
- My Account Login
- Explore content
- About the journal
- Publish with us
- Sign up for alerts
- Open access
- Published: 04 October 2021

AI-based design of a nuclear reactor core
- Vladimir Sobes 1 ,
- Briana Hiscox 2 ,
- Emilian Popov 2 ,
- Rick Archibald 2 ,
- Cory Hauck 2 ,
- Ben Betzler 2 &
- Kurt Terrani 3
Scientific Reports volume 11 , Article number: 19646 ( 2021 ) Cite this article
6881 Accesses
11 Citations
Metrics details
- Applied mathematics
- Computational science
- Energy science and technology
- Engineering
- Mathematics and computing
- Nuclear energy
- Power stations
- Scientific data
The authors developed an artificial intelligence (AI)-based algorithm for the design and optimization of a nuclear reactor core based on a flexible geometry and demonstrated a 3× improvement in the selected performance metric: temperature peaking factor. The rapid development of advanced, and specifically, additive manufacturing (3-D printing) and its introduction into advanced nuclear core design through the Transformational Challenge Reactor program have presented the opportunity to explore the arbitrary geometry design of nuclear-heated structures. The primary challenge is that the arbitrary geometry design space is vast and requires the computational evaluation of many candidate designs, and the multiphysics simulation of nuclear systems is very time-intensive. Therefore, the authors developed a machine learning-based multiphysics emulator and evaluated thousands of candidate geometries on Summit, Oak Ridge National Laboratory’s leadership class supercomputer. The results presented in this work demonstrate temperature distribution smoothing in a nuclear reactor core through the manipulation of the geometry, which is traditionally achieved in light water reactors through variable assembly loading in the axial direction and fuel shuffling during refueling in the radial direction. The conclusions discuss the future implications for nuclear systems design with arbitrary geometry and the potential for AI-based autonomous design algorithms.
Similar content being viewed by others
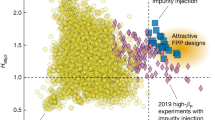
A high-density and high-confinement tokamak plasma regime for fusion energy
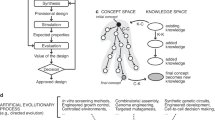
Engineering is evolution: a perspective on design processes to engineer biology
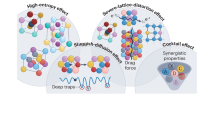
Clarifying the four core effects of high-entropy materials
Introduction.
The rapid development of advanced manufacturing and its application to advanced reactor design in the Transformational Challenge Reactor (TCR) program 1 have presented the opportunity to explore the potential revolutionary benefits of the arbitrary geometry design of nuclear systems. Nuclear engineering design is no longer bound to the simple geometries manufacturable by traditional methods, slabs, cylinders, and spheres (e.g., fuel plates, fuel pellets, fuel pebbles) 2 , 3 . However, the increased freedom of designing an arbitrary geometry system comes at the cost of an increased complexity in design optimization. Dimensionality quickly makes the design problem overwhelming for engineers. To address this issue, the authors implemented an artificial intelligence (AI)-based optimization algorithm and established a challenge problem to demonstrate the application.
The basis of the challenge problem is to determine the optimal geometric shape in the axial dimension of the cooling channels of a simplified reactor design’s full-core model. The reactor core used in this AI design optimization is based on a simplification of the design of the actual TCR core. The core is a right cylinder that is 1 m in diameter and 80 cm tall. Nine concentric rings of hexagonal assemblies surround a central hexagonal assembly. The fuel compact of traditional TRISO particles is an annular design with a 2.5 cm outer diameter, and sits in the middle of each 5 cm wide (flat to flat) hexagonal assembly. There are cooling channels inside the annular fuel, and an yttrium-hydride the moderator surrounds the outside of the fuel annulus. The center assembly in the core is pure moderator, uncooled and unfueled. The helium coolant flows from the bottom to the top in all the fueled assemblies. Figure 1 presents a core schematic.
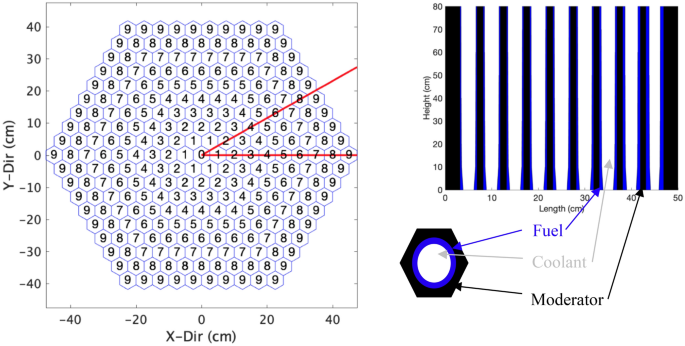
The top view of the core is shown on the left with the nine radial assembly rings labeled. Only the symmetric 1/12 segment inside the red lines is modeled. A horizontal slice of one assembly and an axial cross section of the optimized core configuration is shown on the right. Created using MATLAB R2020b, www.mathworks.com .
The design space encompassed the axial profile of the coolant channel in each of the nine assembly radial rings. That is, all the assemblies in each ring had the same axial coolant channel profile, but the coolant channel profile was different for each radial assembly ring. The geometry optimization was parametrized by a set of coolant channel radii. Each of the nine assembly radial rings had an independent set of nine coolant channel radii which spanned the 80 cm height of the core in 10 cm vertical segments. The radium of the coolant channel was a piece-wise linear function connecting these nine radii for each assembly. The maximum coolant channel radius was constrained to be greater than 1 mm and 2.4 cm (1 mm less than the fuel outer radius).
The objective for this core design was to minimize the temperature peaking factor across each 10 cm vertical segment (eight making the full axial height) of each assembly while maintaining a critical core configuration. The engineering justification for this objective function was to minimize the mechanical stresses due to temperature gradients in the components, although no thermomechanical analyses were performed in this initial study. Specifically, the objective function is defined as:
where \(T\left(x,y,z,\Phi \right)\) is the temperature at the location \(\left(x,y,z\right)\) and design parameters \(\Phi\) .
The challenge problem inherently requires multiphysics modeling between neutron transport and thermofluidics. The problem is further convoluted because the cooling channel radius (axially variable) simultaneously controls everything about the heat transfer process and the amount of fuel at each axial core level because the fuel annulus outer diameter is fixed. The predictive simulation of candidate configurations requires computationally intensive modeling. The authors used a Monte Carlo-based code for neutron transport coupled to a computational fluid dynamics (CFD) code for the thermofluidics. With a large potential design space to explore, it was impractical to evaluate all of the candidate designs with the full fidelity physics. Therefore, the authors developed a machine learning (ML)-based multiphysics emulator that was designed to run on Summit, Oak Ridge National Laboratory’s (ORNL’s) GPU-based high-performance computing (HPC) system 4 . By training the ML-based emulator, the authors achieved errors as low as a few percent, which allowed them to quickly and reliably sample thousands of candidate designs on Summit. Only the most promising candidate designs were tested with the full-fidelity physics simulations. The emulator was updated, and convergence on the optimal design was achieved in only a few iterations.
The “ Background ” section reviews of some of the previous attempts at AI-based nuclear reactor design and optimization that did not leverage the advantages of arbitrary geometry. The section also discusses designing ML-based emulators for computationally intensive full-fidelity modeling software. The “ Methods ” section describes the overall workflow of the authors’ approach and presents the details of the full-fidelity modeling, the design of the emulator, and the optimization approach used on Summit. The “ Results ” section presents and discusses the optimal design solution to the challenge problem. Lastly, the “ Discussion ” section concludes with the authors’ outlook on the future of nuclear systems design in arbitrary geometry and discusses how the AI-based nuclear systems design approach can supplement the toolbox of nuclear industry designers.
Today, reactor cores are built from industrial materials that represent regular (i.e., usually cylindrical or plate) component geometries. The reactor cores have a periodic structure; for example, one component (i.e., core fuel element) is repeated multiple times to create the entire core. This approach results in geometrically similar fuel elements with a regular shape 5 . The fuel volumetric (per unit volume of core) content is spatially uniform. This limitation causes complications and results in fuel usage with different enrichment to efficiently design the entire core, minimizing power and thermal gradients. Similar observations can be made regarding the hydraulic design. The core is cooled to remove heat and generate power. The periodic repeatable fuel structure imminently produces the same regular coolant channel configuration (e.g., all coolant channels are the same and will produce the same level of cooling under the same conditions). The background of this research assumes an additively manufactured core in which the fuel and cooling channel geometries have practically unlimited spatial degrees of freedom. This is the primary difference explored in this work, and it might bring unforeseen performance that could be hidden from designers but easily discoverable for an unbiased AI algorithm.
Previous attempts at AI-based design and optimization of nuclear reactor cores are found in other works; e.g. References 6 , 7 , 8 , 9 , 10 , 11 , 12 , 13 . One common theme among these earlier works is that the optimization problem is posed as a combinatorial problem with fixed geometry rather than as an optimization over continuously variable geometry parametrization. One example is the fuel shuffling during the reloading of a boiling water reactor core. Genetic algorithms have been the predominant choice of AI algorithms for the combinatorial optimization problem. When more continuous variable geometries were studied, more structured optimization approaches were used 14 . A recent work considered the nuclear systems design by using genetic algorithms created from scratch rather than an optimization of an existing configuration 15 . However, arbitrary geometry could only be considered through a voxel representation. Lastly, the authors had published on the framework for an arbitrary geometry optimization of nuclear systems and gave some demonstrations on simplified challenge problems 2 . This work is the application of that framework to a nuclear reactor full core.
The mathematical formulations and their solutions for the underlying multiphysics phenomena that occur in the reactor core are well-known. For this work, the focus in on solutions of the Boltzmann transport equation, which will be the driving term to Poisson’s equation coupled with the Navier–Stokes equations. The solution to this set of coupled equations in nuclear engineering is tied up in several complex computer codes and is computationally intensive. Therefore, an efficient multiparameter optimization search using high-fidelity physics models is prohibitively expensive. In this work, the authors explored the automatic construction of physics-informed ML methods by using emulators with validation from very sparse sampling of the predictive high-fidelity physics simulations. The physics-informed emulators are based on a steady-state reduced-order model through Gaussian kernel convolution that allows for a fast evaluation on a single GPU 16 , which is suitable for ideal scalability in the search over the vast design space.
Surrogate modeling is a well-used method in science and engineering 17 . The fundamental approach is to approximate quantities of interest from complex systems by using cost-effective and accurate surrogates that otherwise could only be measured or simulated at very high cost, if at all. Within the context of design optimization, building cost-effective and accurate surrogates enables the exploration of complicated design space, accelerating the process of finding the best designs for given loss functions. The surrogate modeling method that the authors developed in this work falls under the category of multi-fidelity surrogate modeling. The authors merged the information generated by the dense sampling of reduced-order modeling with sparse full physics simulation via Gaussian processes (GPs) 18 . This work uniquely developed reduced-order models of the neutronics and thermofluidic physics that were designed for a fast evaluation on a single GPU. Thus, it is possible to evaluate millions of designs by using GPU-based HPC systems, such as Summit 4 .
Optimization work flow: inner and outer loops
The authors identified two categories of tasks necessary for successfully developing a holistic AI-based approach to computationally optimizing nuclear systems. The tasks are separated into the inner computational loop and the outer optimization loop. The inner computational loop involves the full-fidelity physics simulation of the candidate designs needed to create the training data for the multiphysics ML-based emulator. The outer optimization loop begins with training the emulator based on the results generated with the full-fidelity physics calculations, and design parameters are chosen from a random sample of the design space. The outer optimization loop is the AI model for the design space that is updated based on massively parallel evaluations of the multiphysics emulator on thousands of candidate geometries. Figure 2 illustrates the workflow. The outer loop can be thought of as an adaptive sampling method, ubiquitous in computation design 17 , where each iteration focuses on a smaller design space that minimizes the given loss function.
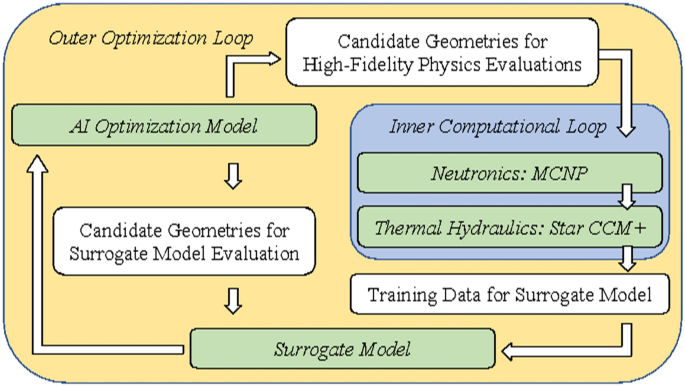
Illustration of the AI optimization workflow. Created using Microsoft PowerPoint, version 16.52, www.microsoft.com .
Inner computational loop
The neutronic modeling for this project was conducted in the Monte Carlo N-Particle code 19 . This code was chosen due to its ability to calculate power in nonfuel materials. This allowed for a very accurate power density profile in all materials. To save on computational time, the model was 30° of the full core (1/12) with reflective boundary conditions (i.e., instead of the full 360°). Because of the intrinsic symmetry and core optimization variables, this model represents a full-core model. The model was run with four tally meshes to account for the energy deposition in a cell from neutrons and photons in the fuel and moderator materials. A tally was produced for each mesh bin. The mesh bin density was 504 in the x direction, 252 in the y direction, and 20 in the z direction. Therefore, the lengths of each mesh bin were 0.1, 0.1, and 4 cm. These sizes were determined because they have a reasonable computational cost neutronically and thermofluidically, and meshing studies determined that they were sufficiently fine to achieve good resolution on the fuel annulus and coolant channels.
There were 750 cycles run with 10,000 particles in each cycle. The first 50 cycles were inactive which means they were not taken into account for the determination of k-effective, flux, or reaction rates. It is necessary to have inactive cycles in the beginning of a Monte Carlo simulation to converge the fission source. The average standard deviation of k-effective was 0.00024 and the maximum was 0.00030. The power density was normalized to maintain a constant core power of 3 MW. MCNP was run in “mode N P” which accounts for neutron-induced photons. This is necessary for the photon-heating tallies.
The thermofluidics model developed for this work relies on numerical methods (e.g., temporal and spatial discretization) and physical models (e.g., turbulent flow, conjugate heat transfer) to predict the temperature and flow distribution in the geometry of interest. The inherent assumptions of these methods and models must be quantified to ensure the correctness and accuracy of the results. In view of the diverse geometry configurations of the optimized designs, an accurate prediction of temperature distribution in the component is necessary. The thermofluidic model must be capable of computing a conjugate (i.e., solid fluid) heat transfer in arbitrary geometric shapes. To achieve this, a CFD approach was taken, and the commercial software STAR-CCM+ was used 20 . This method allows complex surfaces to be discretized with finite volume techniques, as well as allows the interface between the solid structure and the coolant—gas, in this case—to be properly defined. On the solid side, a thermal diffusion of heat with a volumetric heat source is computed to determine the temperature distribution. The heat generated by nuclear fission is deposited in the core element according to the volumetric power distribution supplied by the reactor physics calculation.
On the fluid side, a Reynolds averaging of the velocity vector field was employed within the finite volume formulation. Since the assumed flow is highly turbulent, a two-equation model of turbulence, realizable k-epsilon, was used. This model is better than the standard k-epsilon model for many applications, including rotational and shear flows, and it generally gives answers that are at least as accurate 21 . The near-wall velocity field is resolved with the two-layer all wye (Y) plus method 20 . All these models accurately predict the wall heat transfer, which is critical for the proper resolution of component temperature field.
To model the geometry variation necessary for running the suite of optimization codes, a geometry parameterization method was used. Within the CFD computation, the geometry is regenerated automatically every time a new combination of parameters is tried. The software allows the computational domain to be modified—both in its geometry and discretization—without user intervention. This is achieved by automating the computing process with Java drivers. The approach is fully integrable in an autonomous workflow within the entire optimization suite.
For the thermofluidic calculation, the domain was discretized with a variable resolution but with at least four elements in the radial direction across fuel. It was meshed with a polyhedral mesh with a base dimension determined by the smallest fuel element. Because the geometry varies by fuel and channel sizes, the number of elements per each case is different and usually stays below 7 million. Some specific cases with thin fuel might increase the element count to 20 million, but this is rare. The calculations were run in parallel on 16 processes, and most run time is spent on grid generation. The average clock time for a single run is around 2 h. The cases are run until the convergence of momentum and energy residuals for at least three orders of magnitude is achieved. Sensitivity on mesh and residuals convergence was performed to allow for temperature accuracy of less than 1° to be achieved. The employed modeling approach relies on component physics validation which is part of the software qualification process implemented at ORNL. The flow and heat transfer solutions, and the corresponding modeling techniques, were initially tested and verified by standard test problems. The models will be further tested against integral experiments after such are carried out as part of a comprehensive testing program.
Figure 3 illustrates a typical result from the thermofluidic solution. Contours of temperatures are shown at three axial levels in the core: low (15 cm), middle (45 cm), and top (75 cm). The highest temperature is reached on the fuel-moderator boundary because the design does not involve extra moderator cooling and because the power deposited in the moderator is removed only through fuel-cooling channels. The radial variation of cooling channels is clearly visible, as defined by the optimization algorithm. Channels are larger in the central part and smaller at the periphery where the power density is lower and less coolant is needed. The average pressure drop and fuel temperature and the maximum fuel temperature difference were also calculated and are provided for reference. The input power density is plotted on the left side of Fig. 3 at the same axial positions. The simulation uses power deposition in the fuel and moderator. The power plot gives some impression for the fuel thickness variation (light blue color) with more fuel toward the periphery and less in the middle of core. Although not evident from the limited axial locations plotted, the fuel and channel sizes also vary axially as eight piece-wise linear sections (Fig. 1 ).
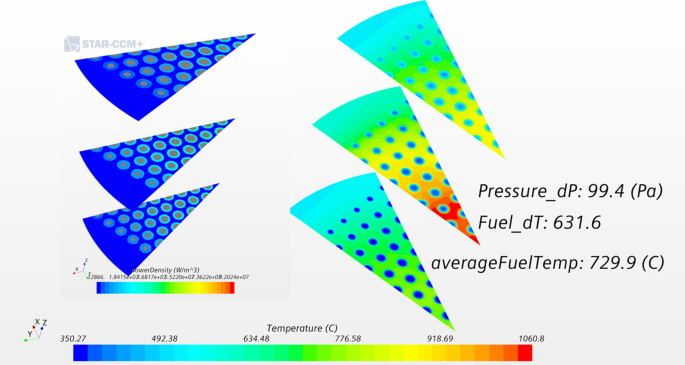
Temperature field from the thermofluidic solution. Three axial sections are plotted at lower, middle, and upper core element levels. They show the temperatures in the coolant, fuel, and moderator. On the left, the power input to the fuel and moderator is provided for reference. Both fuel and channel sizes vary in the radial direction. Created using Star-CCM+, version 2020.1, www.plm.automation.siemens.com/global/en/products/simcenter/STAR-CCM.html .
Outer optimization loop
Simulation-based computational design can quickly become an intractable problem, depending on the size of the computational design space and computational complexity of the design simulation. The authors developed reduced-order surrogate models for neutronics and thermofluidics that can quickly sample hundreds of thousands of geometries on Summit. By using the combination of surrogate modeling and sparse validation with correction from full-physics simulation, the authors were able to train GP ML methods to accurately predict optimal designs. On average, one reactor core design takes ~ 150 s for the reduced-order surrogate model to simulate on a single Summit GPU. This time includes all setup costs and data movement. With six GPUs per node, the authors were able to test ~ 150 reactor geometries per hour per node. Generally, the surrogate is around 95% accurate compared with full physics simulations that use relative least square error measure on the objective function for this problem. Also, the surrogate model generally struggles the most at the inlet region. For the core challenge problem, Summit simulated ~ 10,000 different geometries in combination with ~ 100 full physics simulations in four iterations to determine an optimal design. We provide the code in the supplemental materials for this paper.
The outer loop AI model is based upon Gaussian processes, which are a kernel-based machine learning method that provides an efficient method for ML applicable to physics-oriented problems in engineering sciences. Specifically, given a set,
of training data the loss can be determined for any parameter set. Here, x is the position vector, and \({p}_{i}\) is the parameter vector for the i th design. The functions are the power \({P}_{i}\) , component temperature \({T}_{i}\) , fractional coolant indicator \({V}_{i,c}\) , fractional fuel indicator \({V}_{i,f}\) , and fractional moderator indicator \({V}_{i,m}\) for the N simulated training sets. The loss \({L}_{i}\) for any design is defined to be the standard deviation of temperature for every domain with positive fuel indicator. The last item in this collection is the error estimation of the loss \({\sigma }_{i}\) . The loss of any design \(p\) is predicted by using the kernel-based ML method defined as:
where kernel function, \(k\left(p,{p}_{i}\right)={e}^{-\frac{1}{2}{\| p-{p}_{i}\| }^{2}}\) is used. The coefficients of the kernel-based ML are found by solving
for \(j=1,...,N\) training sets, where L j losses are known. The matrix elements are given as \({K}_{i,j}=k\left({p}_{i},{p}_{j}\right)\) for \(1\le i,j\le N\) and the coefficient vector \(c=\left({c}_{1},...,{c}_{N}\right)\) .
The data from the full-fidelity physic model are augmented with a set of M emulated models:
The ML method assumes that the full fidelity physical models are exact, or \({\sigma }_{i}=0\) for \(i=1,...,N\) . In the case of \(i=N+1,...,N+M\) , a low-resolution approximation of the physics is used to estimate the temperature function \(\tilde{T }\) by solving:
where \({\upsilon }_{i}\left(x\right)\) is a flow field, \(\frac{\partial {\tilde{T }}_{i}\left(x\right)}{\partial x}\) is the temperature gradient along the flow direction, and \({\alpha }_{c}\) , \({\alpha }_{f}\) , and \({\alpha }_{m}\) are constants. For any design \(p\) , the flow field is calculated based on the volumetric rate of coolant. The flow field is zero in the solid material of the reactor. The flow field and constants \({\alpha }_{c}\) , \({\alpha }_{f}\) , and \({\alpha }_{m}\) are calculated such that \({\sum }_{i=1}^{N}{\| {T}_{i}\left(x\right)-{\tilde{T }}_{i}\left(x\right)\| }_{2}\) is minimized, where T i (x) is the training set temperatures.
When the data are augmented, the Gaussian process is calculated by:
where the kernel function, \(k\left(p,{p}_{i}\right)={e}^{-\frac{1}{2}{\| p-{p}_{i}\| }^{2}}\) is used. The coefficients of the kernel-based ML are found by solving
for \(j=1,...,N+M\) and \({\sigma }_{i}=0\) for \(i\le N\) , where the matrix elements are given as \({K}_{i,j}=k\left({p}_{i},{p}_{j}\right)\) for \(1\le i,j\le N+M\) , the coefficient vector \(c=\left({c}_{1},...,{c}_{N+M}\right)\) , and σ i is an estimate for the error in the emulation.
The quantitative objective function for the challenge problem’s optimization design was to minimize the temperature peaking in each 10 cm axial section (eight total for the full-core height) of each assembly in the core. From single-assembly simulations, the average numerical value of the objective function for a conventional design with axially uniform coolant channels of one radius was 842.1 ℃. The optimal design results in a final value of the objective function of 291.35 ℃; a 3× improvement in the objective function is achieved through the AI-based optimization of the geometry of the cooling channels when compared to the constant-cooling-channel-radius design. Figure 4 presents the visualization of the calculated temperature distribution in the core before and after the optimization.
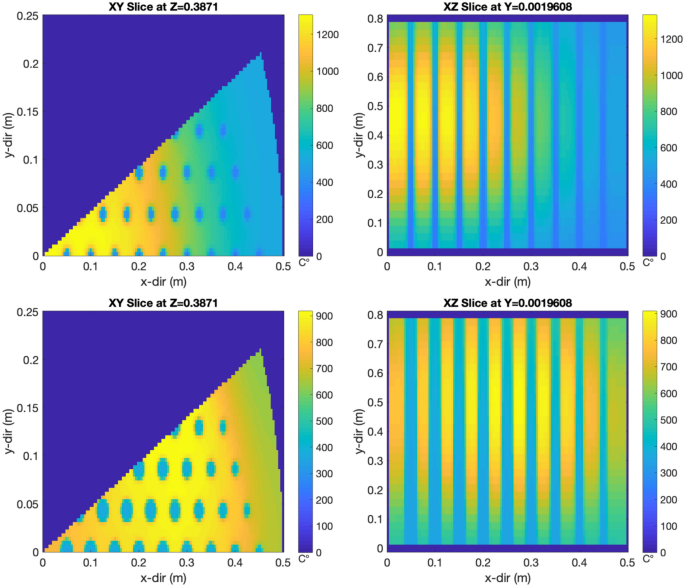
Visual representation of the temperature distribution in the original (top) and optimized (bottom) cores. The left two figures present a radial slice at an axial location of 38.7 cm out of a total core height of 80 cm. The right two figures present an axial slice through the core. Created using MATLAB R2020b, www.mathworks.com .
Figure 5 presents a physical interpretation of the optimization results by plotting the volume of the fuel in each assembly and the heat-exchange area for the cooling channels. The initial configuration with axially uniform cooling channels in all assemblies is given in black. This presents the optimal solution for a uniform cooling channel configuration. An intermediate stage of the optimization process is shown in blue, and the final, optimal design is presented in green. The intermediate result can be identified by the non-smooth behavior of the volume and surface plots across the nine radial assembly rings, whereas the converged solution displays the physically expected smooth behavior. Furthermore, there is a trade-off between the increased heat-exchange area of the cooling channel and the reduced fuel volume. Both contribute in the same direction to reducing the temperature peaking in the middle of the core that is observed with the uniform cooling channel design.
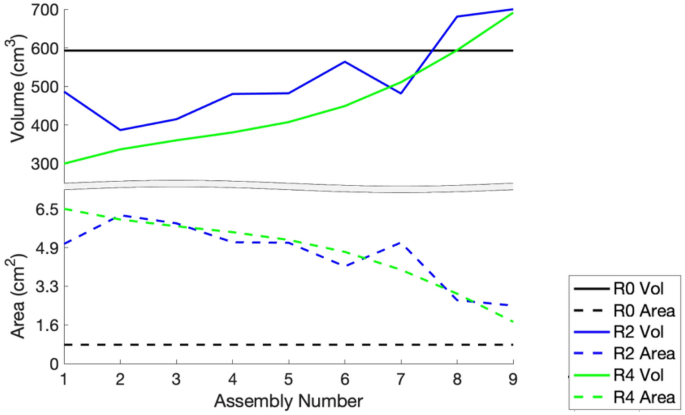
Plot of the fuel volume per assembly (top) and the heat-exchange area for the cooling channel in each assembly (bottom). The three different colors represent different iterations of the optimization algorithm: initial (R0) and two later iterations (R2 and R4). Here, R0, R2, and R4 are shorthand for iteration round zero, two, and four, respectively. Created using MATLAB R2020b, www.mathworks.com .
Although the optimal configuration can be justified from an engineering analysis perspective, the result is far from trivial. The right side of Fig. 1 , which plots the axial cross section of the optimal design, shows that the profile of the cooling channels changes in two dimensions: axially with the height of the core and radially across the different assembly rings. The shape of the cooling channels is also unique for each of the radial assembly rings rather than being a scaled or translated version of each other. The top plot in Fig. 5 also shows a significant reduction in the fuel volume needed to maintain the reactor critical at a fixed power level compared with the traditional, uniform design. Lastly, the extension of this result is that the reactor can be operated at higher power levels for the same amount of fuel and peak fuel temperature limits.
Summary of accomplishments
This paper presents the results for the AI-based design optimization of a full nuclear reactor core with arbitrary geometry cooling channels. To accelerate the optimization space search, the authors developed a ML-based multiphysics emulator capable of running efficiently on Summit. The authors demonstrated how an AI-based optimization algorithm can efficiently sample the vast and continuous search space of arbitrary geometry to find the optimal solution with significant performance improvement. In the established challenge problem, the authors demonstrated a 3× improvement in the performance metric of reducing temperature peaking factors across the core in the radial and axial directions.
Future for nuclear design with arbitrary geometry
The authors envision a rapidly developing and promising future for nuclear systems design with arbitrary geometry. Arbitrary geometry enabled through advanced manufacturing provides a vast variety of unexplored opportunities in nuclear systems design. Historically, many engineering objectives in nuclear reactor design were met through creative combinatorial solutions of fuel loading axially and radially. Arbitrary geometry allows researchers to explore alternative solutions to these engineering challenges. Furthermore, combining variable fuel loading with elements of arbitrary geometry to maximize nuclear reactor safety and economics is an exciting opportunity.
Future for ML-based surrogate models for rapid design evolution
The second exciting opportunity that could result from this work is the rapid design evolution that can be achieved with ML-based surrogate models, as presented in this work. The authors demonstrated that it is possible to construct ML-based surrogate models capable of capturing a large percentage of the system physics but that can be evaluated to predict the performance of candidate designs at a fraction of the computational time. This step is absolutely necessary to progress to searching larger and larger design spaces that allow for more complex nuclear system geometries.
Future for AI-based nuclear design
The authors do not anticipate that the AI-based nuclear systems design will completely replace human designers but rather anticipate that AI-based design will become one of the main tools of the human designer. In this case, the way in which engineers think about the design problem must shift. The new focus must be to carefully craft the parameters of the optimization problem and establish the objective and constraints. The accurate formulation of the objectives will be vital, especially finding the right balance between multiple objectives. The parameters of the optimization must be chosen very carefully to reduce the possible design space as much as possible while maintaining enough flexibility to allow for significant performance improvements.
Although many challenges undoubtedly still remain, the combination of (1) arbitrary geometry enabled by advanced manufacturing, (2) ML-based surrogate models for fast and predictive computational evaluation, and (3) AI-based optimization algorithms form a very exciting future for nuclear design with the potential to discover revolutionary changes in the safety, efficiency, and economy of nuclear systems helping contribute to safer and cleaner energy for the world.
Oak Ridge National Laboratory. Transformational challenge reactor program. https://tcr.ornl.gov/ (2021). Accessed 17 August 2021.
Sobes, V., et al . Artificial intelligence design of nuclear systems empowered by advanced manufacturing. In PHYSOR 2020—Transition to a Scalable Nuclear Future , Cambridge, United Kingdom, March 29–April 2, 2020.
B. R. Betzler, B. J. Ade, et al . Advanced manufacturing for nuclear core design. In PHYSOR 2020—Transition to a Scalable Nuclear Future , Cambridge, United Kingdom, March 29–April 2, 2020.
Oak Ridge Leadership Computing Facility, “Summit: America’s Newest and Smartest Supercomputer,”. https://www.olcf.ornl.gov/Summit/ (2021). Accessed 17 August 2021.
Betzler, B. R. et al. Advanced Manufacturing for Nuclear Core Design (Oak Ridge National Laboratory, 2019).
Book Google Scholar
Wilding, P. R., Murray, N. R. & Memmott, M. J. The use of multi-objective optimization to improve the design process of nuclear power plant systems. Ann. Nucl. Energy 137 , 107079. https://doi.org/10.1016/j.anucene.2019.107079 (2020).
Article CAS Google Scholar
Pereira, C. M. N. A. & Lapa, C. M. F. Coarse-grained parallel genetic algorithm applied to a nuclear reactor core design optimization problem. Ann. Nucl. Energy 30 (5), 555–565. https://doi.org/10.1016/S0306-4549(02)00106-8 (2003).
Pereira, C. M. N. A., Schirru, R. & Martinez, A. S. Basic investigations related to genetic algorithms in core designs. Ann. Nucl. Energy 26 (3), 173–193. https://doi.org/10.1016/S0306-4549(98)00036-X (1999).
Jayalal, M. L., Ramachandran, S., Rathakrishnan, S., Satya Murty, S. A. V. & Sai Baba, M. Application of genetic algorithm methodologies in fuel bundle burnup optimization of pressurized heavy water reactor. Nucl. Eng. Design 281 , 58–71. https://doi.org/10.1016/S0306-4549(98)00036-X (2015).
Pazirandeh, A. & Tayefi, S. Optimizing the fuel management in a VVER-1000 reactor using an artificial neural network. Ann. Nucl. Energy 42 , 112–118. https://doi.org/10.1016/S0306-4549(98)00036-X (2012).
Zameer, A., Mirza, S. M. & Mirza, N. M. Core loading pattern optimization of a typical two-loop 300MWe PWR using simulated annealing (SA), novel crossover genetic algorithms (GA) and hybrid GA(SA) schemes. Ann. Nucl. Energy 65 , 122–131. https://doi.org/10.1016/j.anucene.2013.10.024 (2014).
Gomez-Fernandez, M. et al. Status of research and development of learning-based approaches in nuclear science and engineering: A review. Nucl. Eng. Des. 359 , 110479. https://doi.org/10.1016/j.nucengdes.2019.110479 (2020).
Liu, Z., Wang, J., Tan, S., Qiao, S. & Ding, H. Multi-objective optimal design of the nuclear reactor pressurizer. Int. J. Adv. Nucl. Reactor Design Technol. 1 , 1–9. https://doi.org/10.1016/j.jandt.2019.09.001 (2019).
Article Google Scholar
Betzler, B. R., Chandler, D., Cook, D. H., Davidson, E. E. & Ilas, G. Design optimization methods for high-performance research reactor core design. Nucl. Eng. Des. 352 , 110167. https://doi.org/10.1016/j.nucengdes.2019.110167 (2019).
J. Pevey, O. Chvala, S. Davis, V. Sobes, W.Hines Genetic algorithm design of a coupled fast and thermal subcritical assembly. Nucl. Technol. 206 (4) (2020).
A. Archibald, V. Sobes, B. Hiscox, E. Popov, et al . Physics based machine learning for HPC computational design. In Conference on Data Analysis 2020 , Santa Fe, New Mexico, February 26, 2020.
Forrester, A., Sobester, A. & Keane, A. Engineering Design Via Surrogate Modelling: A Practical Guide (Wiley, 2008).
Williams, C. K. I. & Rasmussen, C. E. Gaussian Processes for Machine Learning Vol. 2 (MIT Press, 2006).
MATH Google Scholar
MCNP—A General Monte Carlo N-Particle Transport Code, Version 5 , Los Alamos National Laboratory, Vol. I, 2–71 ( 2–80 ) (2005).
Siemens. Simcenter STAR-CCM+ software. https://www.plm.automation.siemens.com/global/en/products/simcenter/STAR-CCM.html .
ANSYS, Inc. ANSYS-12.0 Theory Guide (2009).
Download references
Acknowledgements
This manuscript has been authored by UT-Battelle, LLC, under contract DE-AC05-00OR22725 with the US Department of Energy (DOE). The US government retains and the publisher, by accepting the article for publication, acknowledges that the US government retains a nonexclusive, paid-up, irrevocable, worldwide license to publish or reproduce the published form of this manuscript, or allow others to do so, for US government purposes. DOE will provide public access to these results of federally sponsored research in accordance with the DOE Public Access Plan ( http://energy.gov/downloads/doe-public-access-plan ). This work was funded by the U.S. Department of Energy Office of Nuclear Energy Transformational Challenge Reactor program. This research used resources of the Oak Ridge Leadership Computing Facility at the Oak Ridge National Laboratory, which is supported by the Office of Science of the U.S. Department of Energy under Contract No. DE-AC05-00OR22725.
Author information
Authors and affiliations.
University of Tennessee, Knoxville, USA
Vladimir Sobes
Oak Ridge National Laboratory, Oak Ridge, USA
Briana Hiscox, Emilian Popov, Rick Archibald, Cory Hauck & Ben Betzler
Ultra Safe Nuclear Corporation, Oak Ridge, USA
Kurt Terrani
You can also search for this author in PubMed Google Scholar
Contributions
V.S.: conceptualization; formal analysis; investigation; methodology; project administration; supervision; visualization; writing—original draft; writing—review and editing. B.H., E.P.: conceptualization; data curation; formal analysis; investigation; methodology; software; validation; visualization; writing—original draft; writing—review and editing. R.A.: conceptualization; data curation; formal analysis; investigation; methodology; resources; software; validation; visualization; writing—original draft; writing—review and editing. C.H.: conceptualization; formal analysis; investigation; methodology; visualization. B.B., K.T.: conceptualization; funding acquisition; project administration; resources; supervision; writing—review and editing.
Corresponding author
Correspondence to Vladimir Sobes .
Ethics declarations
Competing interests.
The authors declare no competing interests.
Additional information
Publisher's note.
Springer Nature remains neutral with regard to jurisdictional claims in published maps and institutional affiliations.
Supplementary Information
Supplementary information., rights and permissions.
Open Access This article is licensed under a Creative Commons Attribution 4.0 International License, which permits use, sharing, adaptation, distribution and reproduction in any medium or format, as long as you give appropriate credit to the original author(s) and the source, provide a link to the Creative Commons licence, and indicate if changes were made. The images or other third party material in this article are included in the article's Creative Commons licence, unless indicated otherwise in a credit line to the material. If material is not included in the article's Creative Commons licence and your intended use is not permitted by statutory regulation or exceeds the permitted use, you will need to obtain permission directly from the copyright holder. To view a copy of this licence, visit http://creativecommons.org/licenses/by/4.0/ .
Reprints and permissions
About this article
Cite this article.
Sobes, V., Hiscox, B., Popov, E. et al. AI-based design of a nuclear reactor core. Sci Rep 11 , 19646 (2021). https://doi.org/10.1038/s41598-021-98037-1
Download citation
Received : 07 April 2021
Accepted : 02 September 2021
Published : 04 October 2021
DOI : https://doi.org/10.1038/s41598-021-98037-1
Share this article
Anyone you share the following link with will be able to read this content:
Sorry, a shareable link is not currently available for this article.
Provided by the Springer Nature SharedIt content-sharing initiative
This article is cited by
Dynamic recrystallization behavior and strengthening mechanism of a novel mo–ti3alc2 alloy at ultrahigh temperature.
- Xin-Yuan Zheng
- Shi-Zhong Wei
Rare Metals (2024)
By submitting a comment you agree to abide by our Terms and Community Guidelines . If you find something abusive or that does not comply with our terms or guidelines please flag it as inappropriate.
Quick links
- Explore articles by subject
- Guide to authors
- Editorial policies
Sign up for the Nature Briefing: AI and Robotics newsletter — what matters in AI and robotics research, free to your inbox weekly.

Laying the Foundation for New and Advanced Nuclear Reactors in the United States
NATIONAL ACADEMIES OF SCIENCES, ENGINEERING, AND MEDICINE NATIONAL ACADEMY OF ENGINEERING
The world confronts an existential challenge in responding to climate change, resulting in an urgent need to reduce greenhouse gas emissions from all sectors of the economy.
What will it take for new and advanced nuclear reactors to play a role in decarbonization? Nuclear power provides a significant portion of the world’s low-carbon electricity, and advanced nuclear technologies have the potential to be smaller, safer, less expensive to build, and better integrated with the modern grid. However, if the United States wants advanced nuclear reactors to play a role in its plans for decarbonization, there are many key challenges that must be overcome at the technical, economic, societal, and regulatory levels.
A new National Academies’ report, Laying the Foundation for New and Advanced Nuclear Reactors in the United States , discusses how the United States could support the successful commercialization of advanced nuclear reactors with near-term actions to establish policies and practices.
Nuclear Power and the Changing Energy Landscape
The U.S. electric power system is undergoing a sweeping transition as the nation moves to reduce emissions and prevent the worst effects of climate change. Plans for decarbonization rely heavily on powering as many industries as possible with low-carbon energy, from transportation to manufacturing. Meeting the growing demand for low-carbon electricity in the future will be a key challenge to overcome for the United States and the world.
If the United States wants nuclear power as part of its future low-carbon energy landscape, it will be important to provide support for the demonstration, commercialization, and deployment of new and advanced nuclear reactors.
18.2% U.S. ELECTRICITY
Nuclear power plants provide half of all low-carbon electricity in the United States. They supply 18.2% of the nation’s total electricity.
92 POWER PLANTS
There are 92 nuclear power plants in operation in the United States (with 442 across the globe).
The initial licensing lifetime of a nuclear power plant in the United States is 40 years.
Demand for low-carbon electricity is projected to grow significantly.
Despite uncertainty about the level of future demand, there are likely to be many opportunities for low-carbon energy technologies such as advanced nuclear.
Projected future electricity demand across key sectors. Three different studies show low, medium, and high growth in demand. Source: NREL 2022
What are advanced nuclear reactors and how can they be used?
Light water reactors (LWRs) are the only type of reactor currently in use at commercial nuclear power plants in the United States. However, many companies are working to develop new small modular LWR designs as well as advanced reactor concepts that are fundamentally different from the LWR design. These new and advanced nuclear reactors could potentially meet a much wider variety of energy needs than the light water reactors in service today. These applications could include:
- GENERATING ELECTRICITY FOR THE GRID
- PROVIDING HEAT
- PROVIDING PORTABLE POWER

GENERATING ELECTRICITY FOR THE GRID The main use for advanced nuclear reactors moving forward is likely to be as small modular reactors producing electricity for the grid . Some smaller reactors could be manufactured in a controlled factory setting to reduce on-site construction costs. These new power plants could potentially repurpose fossil fuel plants and take advantage of existing infrastructure.

Advanced nuclear reactors could provide heat for:
- Thermal energy storage for electricity production
- High-temperature heat for industry , such as chemical processing or hydrogen production
- Low-temperature heat for district heating, desalination, or agriculture

Advanced nuclear reactors could provide portable power for:
- Microreactors (1-10 MWe) for remote sites or transportable microreactors to meet emergency needs
- Marine propulsion
- Remote military bases
How could advanced nuclear reactors fit into decarbonization moving forward?
The race against climate change is both a marathon and a sprint. Growth in electricity demand and the need to achieve economy-wide decarbonization over the coming several decades present important long-term opportunities for advanced nuclear technologies.
The earliest demonstrations of advanced nuclear reactors are likely to be complete in the 2030s, but the timing of widescale deployment depends on many issues, especially market competitiveness.
Timeline for potential technology demonstrations and commercialization of some advanced nuclear reactors alongside broader decarbonization efforts.
- Recommendations
Developing advanced reactors on a timeframe to significantly contribute to a decarbonized energy system will require sustained effort and robust financial support in this decade and beyond by the U.S. government, the nuclear industry, and the financial community. The successful commercialization of advanced reactors will require:
For the complete list of findings and recommendations, see Appendix I of the report.
Closing Technology Research Gaps
Advanced nuclear reactor technologies hold the promise for safer, more efficient, and more nimble designs than currently deployed nuclear technologies. However, the various advanced reactors under development are at different levels of technological maturity. These projects must resolve technology gaps and demonstrate new business use cases before wide-scale deployment.
While many of the current concepts plan to move towards commercial reactor demonstration with existing materials, focused investment to create better-performing materials (particularly for reactor core materials and cladding) could lead to significant improvements in safety, reliability, and affordability.
RECOMMENDATION 2.2: The Department of Energy’s Office of Nuclear Energy should initiate a research program that sets aggressive goals for improving materials performance. This could take the form of a strategic partnership for research and development involving NRC, EPRI, the nuclear industry, national labs, and universities. The program should incentivize the use of modern materials science to decrease the time to deployment of materials with improved performance and to accelerate the qualification (ASME Section III, Division 5 or equivalent) and understanding of life-limiting degradation processes of a limited number of high performance structural materials, e.g., reactor core materials and cladding. Learn more in Chapter 2 .
In addition to providing electricity to customers across economic sectors, nuclear power plants can provide heat for industrial processes. The heat generated could be used for desalination, district heating, or producing hydrogen or synthetic fuels. All of these applications could become important as the chemical, materials, and transportation sectors transition to low-carbon operations.
RECOMMENDATION 5.1: Industrial applications using thermal energy present an important new mission for advanced reactors. Key research and development needs for industrial applications include assessing system integration, operations, safety, community acceptance, market size as a function of varying levels of implicit or explicit carbon price, and regulatory risks, with hydrogen production as a top priority. The Department of Energy, with the support of industry support groups such as the Electric Power Research Institute and the nuclear vendors, should conduct a systematic analysis of system integration, operation, and safety risks to provide investors with realistic models of deployment to inform business cases and work with potential host communities. Learn more in Chapter 5 .
Structured Federal Funding from Design to Deployment
In order to ensure the efficient deployment of scarce resources, U.S. federal government programs for advanced nuclear development need better coordination and continuity from early research and development through demonstration and deployment. Programs should include decision points for continuation or termination of funding for specific reactor concepts dependent on meeting key milestones for performance, budget, and siting.
RECOMMENDATION 4.2: The nuclear industry and the Department of Energy’s Office of Nuclear Energy should fully develop a structured, ongoing program to ensure the best performing technologies move rapidly to and through demonstration as measured by technical (testing, reliability), financial (cost, schedule), regulatory, and social acceptance milestones. Concepts that do not meet their milestones in the ordinary course should no longer receive support and newer concepts should be allowed to enter the program in their place.
RECOMMENDATION 4.3: Congress and the DOE should maintain the Advanced Reactor Demonstration Program concept. The DOE should develop a coordinated plan among owner/operators, industry vendors, and the DOE laboratories that supports needed development efforts. The ARDP plan needs to include long-range funding linked to staged milestones; on-going design, cost, and schedule reviews; and siting and community acceptance reviews. This plan will help DOE downselect among concepts for continued support toward demonstration. A modification in the demonstration schedule that takes a phased (vs. concurrent) approach to reactor demonstration may be required. For example, funding would be continued for the first two demonstrations under the ARDP. A second round of demonstrations of designs expected to mature from the current ARDP Risk Reduction for Future Demonstrations award recipients could be funded for demonstration under an “ARDP 2.0” starting thereafter and going into the future. Learn more in Chapter 4 .
Improved Project Management and Construction
Nuclear projects in the U.S. and Europe have not been built on budget or on schedule in recent decades. These cost overruns are due to a variety of factors, including that a typical U.S. utility company does not have adequate technical and engineering personnel to plan and manage a nuclear construction project. Expertise and resources for project management are needed to support and streamline power plant construction.
Much of the cost growth does not necessarily arise from the nuclear island , but from the civil works (e.g., concrete or steel structures and the rest of the power plant).

RECOMMENDATION 6.9: While it is vital to demonstrate that advanced reactors are viable from a technical perspective, it is perhaps even more vital to ensure that the overall plant, including the on-site civil work, can be built within cost and schedule constraints. Since it is likely that costs for onsite development will still be a significant contributor to capital cost, and the ~$35M in DOE funding for advanced construction technologies R&D is small in comparison to the hundreds of millions spent on nuclear island technology research, more should be done over an extended period to research technologies that may streamline and reduce costs for this work. Learn more in Chapter 6
Some advanced reactor vendors are considering moving from the traditional “project-based” approach to a “product-based” approach in which the reactor is produced in a factory or shipyard, with the goal of improving schedules and quality while reducing costs and construction risks. However, even if this method improves the construction of nuclear components, extensive on-site construction work will still remain for the civil works.
RECOMMENDATION 6.2: Nuclear owner/operators pursuing new nuclear construction should consider establishing a consortium or joint venture to pursue the construction on behalf of the group, thereby enabling the creation and maintenance of the necessary skilled personnel to pursue projects successfully. Alternatively, advanced reactor developers operating within the traditional project delivery model should implement a long-term business relationship, preferably an equity partnership such as a joint venture or a consortium, with a qualified engineering, procurement, and construction (EPC) firm experienced in the nuclear industry. Learn more in Chapter 6 .
Strengthening the Skilled Workforce
Nuclear energy technologies require a highly skilled workforce, and the process to support and sustain these technologies over their 60+ year service life is complex and expensive. In addition to the staff needed to develop and build the next-generation of nuclear power plants, there must also be a training pipeline for staff to service the plants over many decades; technical experts to manage the fuel cycle; and regulatory, legal, and policy experts to handle licensing and oversight.
RECOMMENDATION 6.1: In anticipation of the necessary expansion in workforce to support more widespread deployment of nuclear technologies, the Department of Energy should form a cross-department (whole of government) partnership to address workforce needs that is comparable to initiatives like the multi-agency National Network for Manufacturing Innovation. The program would include the Departments of Labor, Education, Commerce, and State, and would team with labor organizations, industry, regulatory agencies, and other support organizations to identify gaps in critical skills and then fund training and development solutions that will close these gaps in time to support more rapid deployment. Learn more in Chapter 6 .
Timely Updates to Regulations
Domestic power reactors are tightly regulated by the U.S. Nuclear Regulatory Commission (NRC) in all phases of their lifecycle, including design, construction, operations, and decommissioning. The NRC is tasked with protecting public health, safety, and the environment by adjusting regulatory requirements and verifying safety claims for new reactor technologies and applications. Advanced nuclear reactors present new use cases and regulatory challenges. Work to develop new regulatory frameworks should begin now to support future deployment of advanced nuclear reactors.
While the NRC must maintain its overriding commitment to safety, the regulatory process should be made as efficient as possible if advanced reactors are to be commercialized in the coming decades.
RECOMMENDATION 7.1: Advanced reactors will not be commercialized if the regulatory requirements are not adjusted to accommodate their many differences from existing light water reactors. A clear definition of the regulatory requirements for a new technology must be established promptly if timely deployment is to be achieved. The NRC needs to enhance its capability to resolve the many issues with which it is and will be confronted. In recognition of the urgency for the NRC to prepare now, Congress should provide increased resources on the order of 10s of millions of dollars per year to the NRC that are not drawn from fees paid by existing licensees and applicants. Learn more in Chapter 7
Some reactor vendors anticipate opportunities to deploy their reactors near or in urban environments or in the vicinity of industrial facilities that will use heat produced by the reactor. These applications of advanced reactors will present unique siting and emergency planning issues. Careful and early examination of such issues is necessary to define the future range of economic opportunities that are available for advanced reactors.
RECOMMENDATION 7.4: The NRC should expedite the requirements and guidance governing siting and emergency planning zones (EPZs) in order to enable vendors to determine the restrictions that will govern the deployment of their reactors. Learn more in Chapter 7 .
Community Engagement and Societal Acceptance
Societal acceptance is necessary if new reactors are to play an expanded role in a decarbonized energy system, and it should be considered early in the design and verification process. The industry should engage with communities affected by prospective new construction, hear their needs and concerns, and adjust plans as a result. The effort should reflect an overriding commitment to honesty, early engagement through credible information channels, and genuine effort to develop a partnership.
Sociological approaches must become part of the nuclear energy research and development cycle, treated with the same seriousness as technology development. New risk communication strategies—grounded in rigorous social science (rather than polling) and respect for community apprehensions and desires—could greatly improve the prospects for nuclear deployment in the coming decades.
RECOMMENDATION 8.4: The developers and future owners that represent the advanced nuclear industry must adopt a consent-based approach to designing, siting, and operating new facilities. The siting approach will have to be adjusted for a particular place, time, and culture. The nuclear industry should follow the best practices, including:
Following these practices will require additional time and financial resources to be allotted to successfully site and construct new nuclear power facilities, and the industry must account for these costs in their plans. The industry should be willing to fully engage with a community, hear their concerns and needs and be ready to address them, including adjusting plans. The industry, guided by experts in consent-based processes, should capture best siting practices in guidance documents or standards. The study held an information gathering workshop on understanding societal challenges facing nuclear power in September 2021. Watch videos from the talks and explore the topics discussed on the workshop resource page . Learn more in Chapter 8 .
Advancing Security and Safeguards
Advanced reactor designs and deployment scenarios are far different from conventional nuclear power reactors, with a different set of security concerns. New security considerations for advanced nuclear reactor designs include:
The Nuclear Regulatory Commission (NRC) has proposed significant modifications to physical security requirements to accommodate designs and operations proposed by licensees of advanced reactors. There are many hurdles remaining. Clear NRC guidance is needed, as well as a fuller understanding of the vulnerabilities that the new designs and deployment scenarios may present.
RECOMMENDATION 9.1: The modification of the security requirements proposed by the NRC staff could have significant implications for the design, staffing and operations of advanced reactors, thereby impacting business plans. Delays in providing clear regulatory guidance may impact capital availability and increases the potential for costly re-design if guidelines do not align with expected modifications to existing protocols. Congress should provide additional funding for NRC evaluation of security guidelines and the Commission should expedite its consideration of the staff proposal and seek to complete the rulemaking promptly if significant changes are deemed appropriate. In that case, the prompt completion of the associated guidance should also be a high priority. Learn more in Chapter 9
As advanced reactors continue to be developed with the potential of rapid scale-up both domestically and internationally in the coming decades, it is crucial to recognize, prioritize, and address gaps in safeguards technology and to incorporate key measurement capabilities at the earliest stages of the design process. The U.S. government has established a robust set of programs and organizations that will support advanced reactor developers across the spectrum of research, development, and deployment, including support for domestic and international safeguards and security research, international engagement, and licensing assessment.
RECOMMENDATION 9.4: The United States should increase sustained long-term financial and technical support for bilateral and multilateral programs (e.g., IAEA) to build capacity in likely partner countries to deploy new and advanced reactors that meet safety, security and safeguards requirements, including support from U.S. national labs and universities as training platforms. It should seek commitments from vendors, supplier countries, and customer countries to adhere to the highest standards of safety, safeguards, and security. The Departments of Energy and State should partner with U.S. reactor vendors to develop a safety, safeguards and security “package,” where the United States and the vendor could offer customized support to a host country for developing and implementing new safety, security and safeguards arrangements. Learn more in Chapter 9 .
Developing Competitive Financing Options
The upfront financing costs for developing nuclear reactors are currently higher than those for other energy technologies because of large capital requirements, extended development timelines, and limited financing options. Incentives to support commercialization and more flexible financing options would help support new reactor deployment.
The commercial deployment of low-carbon energy resources will require substantial investment. In order to obtain funds at this scale, the investments must present sufficiently low risk that they can compete with other “ordinary” investments in the public equity and debt markets. Widespread commercial deployment of nuclear reactors will occur only if the projects can convincingly demonstrate that they can compete in a marketplace with alternatives.
RECOMMENDATION 4.4: To enable a cost-competitive market environment for nuclear energy, federal and state governments should provide appropriately tailored financial incentives (extending and perhaps enhancing those provided recently in the Inflation Reduction Act) that industry can use as part of a commercialization plan, consistent with the successful approaches used with renewables. These tools may vary by state, locality, and market type. Continued evaluation of the recently passed incentives will need assessment to determine their adequacy. The scale of these incentives needs to be sufficient not only to encourage nuclear vendors, but also the supporting supply chains. Learn more in Chapter 4
Many new and advanced nuclear vendors in the United States anticipate a strong international market for their designs. However, non-U.S. vendors have more options for financing the export and deployment of advanced reactors than U.S. vendors, which will reduce the competitiveness of U.S. companies and limit opportunities for the United States to promote global nuclear safety and security.
RECOMMENDATION 10.2: International nuclear projects by U.S. exporters are likely to require a financing package that reflects a blending of federal grants, loans, and loan guarantees along with various forms of private equity and debt financing. The Executive Branch should work with the private sector to build an effective and competitive financing package for U.S. exporters. Learn more in Chapter 10 .
Committee Members

Richard A. Meserve, Chair

Ahmed Abdulla

Jaquelin Cochran

Michael L. Corradini

Richard Cupitt

Leslie Dewan

Michael Ford

Kirsty Gogan

Allison M. Macfarlane

David K. Owens

James Rispoli

Sola Talabi

Steven J. Zinkle
Dr. Ahmed Abdulla is an assistant professor in the Department of Mechanical and Aerospace Engineering at Carleton University (Ottawa, ON). He develops energy system models for deep decarbonization. Modeling efforts focus on evaluating the role of disruptive energy technologies that sit at a low level of technical readiness; including energy storage systems, advanced nuclear power, and negative emissions technologies. Dr. Abdulla advances process modeling, systems engineering, engineering economics, and quantitative risk and decision analysis in his research. He integrates insights from public policy and behavioral science in his models, to deploy truly sustainable technologies—ones that are both techno-economically viable and socio-politically acceptable. Dr. Abdulla co-leads the APEX (Alternative Pathways for the Energy Transition) research group at Carleton, which consists of highly interdisciplinary engineers devoted to accelerating the transition to a deeply decarbonized energy system and averting the worst consequences of climate change. Results from his research have been published in leading journals, including Nature Climate Change, Nature Communications, the Proceedings of the National Academy of Sciences, Philosophical Transactions of the Royal Society; Environmental Science and Technology; Risk Analysis; and Environmental Research Letters. His findings have been featured in The Wall Street Journal, The Los Angeles Times, Bloomberg News, and National Public Radio. He received his B.S.E. in Chemical Engineering from Princeton University, and his Ph.D. in Engineering and Public Policy from Carnegie Mellon University.
Dr. Todd Allen is currently a faculty member and chair of the Nuclear Engineering & Radiological Sciences Department at the University of Michigan and a senior fellow at Third Way, a DC-based think tank, supporting their clean energy portfolio. He was the Deputy Director for Science and Technology at the Idaho National Laboratory from January 2013 through January 2016. Both the INL and Third Way positions occurred while on leave from the University of Wisconsin. Previously, he was a professor in the Engineering Physics Department at the University of Wisconsin, a position held from September 2003 through December 2018. In addition to his teaching and research responsibilities at Wisconsin, he was also the Scientific Director of the Advanced Test Reactor National Scientific User Facility, centered in Idaho Falls, Idaho, at the Idaho National Laboratory. He held that position from March 2008-December 2012. He was also the Director of the Center for Material Science of Nuclear Fuel, a Department of Energy-sponsored Energy Frontier Research Center. Prior to joining the faculty at the University of Wisconsin, he was a Nuclear Engineer at Argonne National Laboratory-West in Idaho Falls. His doctoral degree is in Nuclear Engineering from the University of Michigan (1997). Prior to graduate work, he was an officer in the United States Navy Nuclear Power Program.
Dr. Jaquelin Cochran is the director of the Grid Planning and Analysis Center at the National Renewable Energy Laboratory, where she has worked since 2009. Dr. Cochran’s work has focused on the evolution of the power grid with high deployment of renewable energy. She recently led the Los Angeles 100% Renewable Energy Study and a portfolio of analyses about India’s power system. Before joining NREL, Dr. Cochran was an Assistant Professor of Natural Resource Management with KIMEP University in Almaty, Kazakhstan. She also served as a Peace Corps Volunteer for two years with the Polish Foundation for Energy Efficiency (FEWE) in Krakow. She holds a Ph.D. and M.A. from the Energy & Resources Group at the University of California at Berkeley, and a B.A. from Pomona College.
Dr. Michael L. Corradini (NAE) is Emeritus Wisconsin Distinguished Professor of Nuclear Engineering and Engineering Physics at the University of Wisconsin-Madison. He served from 1995 to 2001 as Associate Dean for the College of Engineering and as Chair of Engineering Physics from 2001-2011. He has published widely in areas related to vapor explosion phenomena, jet spray dynamics, and transport phenomena in multiphase systems. From 1978-1981 he served as a member of technical staff of Sandia National Laboratories. In 1998, he was elected to the National Academy of Engineering. He has also served as a presidential appointee in 2002 and 2003 as the chairman of the Nuclear Waste Technical Review Board (a separate government agency). From 2004-2008, he served as a board member of the INPO National Accreditation Board for Nuclear Training. In 2006, he was appointed to the NRC Advisory Committee on Reactor Safeguards and was elected to the National Council on Radiation Protection. In 2010, he was appointed Chair of the Scientific Advisory Committee to the French Atomic Energy Agency. He began and served as the Director of the Wisconsin Energy Institute. He was elected as the President of the American Nuclear Society for 2012 – 2013. Michael received his B.S. in Mechanical Engineering from Marquette University, Milwaukee WI; M.S. in Nuclear Engineering from Massachusetts Institute of Technology, and Ph.D. in Nuclear Engineering from Massachusetts Institute of Technology.
Dr. Richard Cupitt is a senior fellow and director of the Partnerships in Proliferation Prevention program at Stimson. His areas of expertise include WMD nonproliferation, export controls, and foreign policy. Prior to joining Stimson, he served as the Special Coordinator for U.N. Security Council resolution 1540 in the Office of Counterproliferation Initiatives at the U.S. State Department from 2012 through 2016. As such, he led U.S. government efforts to further implementation of the more than two hundred legally binding obligations and recommendations of the resolution, which aims to combat proliferation of WMD and their means of delivery, especially to non-state actors such as terrorists and criminal organizations. From 2005 to 2012, he worked as an expert for the committee established pursuant to U.N. Security Council resolution 1540 (2004), a subsidiary body of the U.N. Security Council, monitoring and facilitating implementation of the resolution in all U.N. Member States, along with building relationships with more than forty international organizations, coordinating assistance activities, and conducting outreach with industry and academia. Elected coordinator of the experts from 2010-2012, he also led the work in several specialized areas including combating the financing of proliferation and export controls. From 2004 to 2008, Cupitt also held a position as Scholar-in-Residence at American University and worked as Special Adviser for International Cooperation for the U.S. Undersecretary of Commerce in the Bureau of Industry and Security from 2002-2004. From 1988 to 2002, Cupitt had various posts for the Center International Trade and Security (CITS) of the University of Georgia, including Associate Director, as well as acting as a visiting scholar at the Center for Strategic and International Studies (CSIS) in 2000-2002. Cupitt also has held academic positions at Emory University and the University of North Texas. He has produced four books and more than 20 peer-reviewed articles on nonproliferation export controls, along with dozens of other security or trade-oriented publications. In addition, he has served as a consultant on projects for the U.S. State Department, several U.S. national commissions, U.S. national nuclear laboratories, and various international organizations.
Dr. Leslie Dewan is the CEO of RadiantNano, a nuclear startup developing next-generation radiation detectors with applications in national security, clean energy production, and medical diagnostics. Previously, she was the CEO of Transatomic Power, a company that designed safer nuclear reactors that leave behind less waste than conventional designs. Leslie received her Ph.D. in nuclear engineering from MIT, with a research focus on computational nuclear materials. She also holds S.B. degrees from MIT in mechanical engineering and nuclear engineering. Before starting her Ph.D., she worked for a robotics company in Cambridge, MA, where she designed search-and-rescue robots and equipment for in-field identification of chemical and nuclear weapons. Leslie has been awarded an MIT Presidential Fellowship and a Department of Energy Computational Science Graduate Fellowship. She has served on the MIT Corporation, MIT’s board of trustees. Leslie has been named a TIME Magazine "30 People Under 30 Changing the World," an MIT Technology Review "Innovator Under 35," a Forbes "30 Under 30,” a National Geographic Explorer, and a World Economic Forum Young Global Leader.
Dr. Michael Ford is the Associate Laboratory Director for Engineering at the Princeton Plasma Physics Laboratory. In this role, Dr. Ford leads the pursuit of PPPL’s mission to develop advanced fusion engineering knowledge and techniques and is responsible for all engineering support throughout the Laboratory. Prior to assuming his position at PPPL, Dr. Ford served as the Strategy Development Director for the Energy and Global Security (EGS) Directorate at the Argonne National Laboratory. At Argonne, he helped develop strategies designed to build increased sponsor support for energy and national security-related research. Dr. Ford remains active in energy, engineering risk, and environmental policy research, and led Phase I of the National Demonstration Reactor Siting Study supporting the National Reactor Innovation Center. Prior to his work in the National Laboratories system, Dr. Ford held research positions at the Harvard University Center for the Environment and at the Belfer Center for Science and International Affairs, Harvard Kennedy School. He earned his PhD in engineering and public policy at Carnegie Mellon University (CMU), where he conducted research in energy and the environment, with a focus on advanced reactor technology development and proliferation risk. Dr. Ford also served a full career as an officer in the United States Navy and held Navy subspecialties in nuclear engineering, resource management, and operations analysis. During his time on active duty, CAPT (Ret) Ford commanded the guided-missile cruiser USS BUNKER HILL (CG 52) and the guided-missile destroyer USS MUSTIN (DDG 89) and served as lead nuclear engineer (Reactor Officer) aboard USS NIMITZ (CVN 68). Ashore, he held senior finance and resource management positions on the U.S. Navy and U.S Joint Staffs at the Pentagon. In these positions, he developed standards for new warfare systems development and helped lead the Navy Quadrennial Defense Review process.
Kirsty Gogan is an internationally sought-after advisor to governments, industry, academic networks and NGOs. Kirsty is regularly invited as an expert speaker on science communication, climate change, competitiveness and innovation to high profile events around the world. She has more than 15 years of experience as a senior advisor to Government on climate and energy policy, including 10 Downing Street, and the Office of the Deputy Prime Minister. As Deputy Head of Civil Nuclear Security, Kirsty reviewed the UK national communications response to Fukushima, and implemented a number of recommendations supported by industry and Government. She subsequently provided editorial oversight for the revision of the Civil Nuclear Emergency Planning and Response Guidance. Leading the Government’s public consultation into the UK’s nuclear new build program, Kirsty addressed public concerns about nuclear power and engaged antinuclear campaigners in a constructive dialogue process with Government that continues to this day. Kirsty is now managing partner of LucidCatalyst, a highly specialized international consultancy offering thought leadership, strategy development and techno-economic expertise focused on multiplying and accelerating zero carbon technology options available for large-scale, affordable, market-based decarbonization of the global economy over a wide range of future scenarios. Kirsty is also co-founder of Energy for Humanity (EFH), an environmental NGO focused on large scale deep decarbonization and energy access. Under Kirsty’s leadership, EFH was shortlisted for the Business Green Leaders “Green NGO of the Year” Award in 2016 and received the U.S. Nuclear Industry Council Trailblazer Award in 2019. At COP21 in Paris, EFH organized a press conference that led to media coverage reaching an estimated audience of 800 million people globally. EFH jointly launched the Clean Energy Ministerial Flexible Nuclear Campaign in May 2019, supported by the Canadian, U.S., and UK governments, and in partnership with ClearPath Foundation. Kirsty is also co-founder, with Eric Ingersoll, of TerraPraxis, a new non-profit focused on energy innovation for a prosperous planet.
Dr. Ning Kang is the Department Manager of the Idaho National Laboratory’s Power & Energy Systems Department, specializing in modeling of distributed energy resources (DERs), microgrid design and field deployment, hydropower analysis, clean and renewable energy resources integration into the grid, power and controller hardware-in-the-loop testing, development of intermediate-temperature and high-temperature electrolysis, and integration of hydrogen production with nuclear power plants. Before joining INL, she was a Principal Engineer at the Argonne National Laboratory where she focused on research in transmission and distribution systems (T&D) co-simulation and planning and operations coordination, reliability impact of DERs on the bulk electric system (BES), advanced distribution management system (ADMS), and microgrid integration and impact analysis. Ning has had 6 years of industry R&D experience when she was a Senior R&D Engineer at ABB U.S. Corporate Research Center in Raleigh, NC. At ABB, she was the main contributor to developing a substation-based protection and automation solution, a Volt-VAR optimization solution considering high DER penetration for ABB’s DMS software, and condition-based and data-driven algorithms for ABB’s recloser controllers. She is the main creator of an open-source T&D systems co-simulation software (TDcoSim) and holds five U.S. patents and one U.S. patent application. She has co-authored more than 39 journal and conference papers, book chapters, and technical reports. Ning is a senior member of the IEEE Power and Energy Society. She is also a member of NERC’s Systems Planning Impacts of Distributed Energy Resources Working Group (SPIDERWG). Dr. Kang currently serves as the senior editor of the Power and Energy Section of the IEEE Access Journal and the steering committee member of IEEE Transactions on Cloud Computing. Dr. Kang has been a registered Professional Engineer since 2015. She earned her doctorate in electrical engineering from the University of Kentucky.
Dr. Allison M. Macfarlane is currently Professor and Director, School of Public Policy and Global Affairs, Faculty of Arts, UBC. Dr. Macfarlane has held both academic and government positions in the field of energy and environmental policy, especially nuclear policy. Most recently, she directed the Institute for International Science and Technology Policy at the George Washington University. She recently held a fellowship at the Wilson International Center for Scholars in Washington, DC and was Fulbright Distinguished Chair in Applied Public Policy at Flinders University and Carnegie Mellon Adelaide in Australia. The first geologist (and the third woman) to chair the U.S. Nuclear Regulatory Commission from 2012-2014, Dr. Macfarlane holds a doctorate in earth science from MIT and a bachelor's of science from the University of Rochester. She has held fellowships at Radcliffe College, MIT, Stanford, and Harvard Universities, and she has been on the faculty at Georgia Tech in Earth Science and International Affairs, at George Mason University in Environmental Science and Policy, and in the Elliott School of International Affairs at George Washington University. From 2010 to 2012 Dr. Macfarlane served on the White House Blue Ribbon Commission on America's Nuclear Future, created by the Obama Administration to recommend a new national policy on high-level nuclear waste. She has also served on National Academy of Sciences panels on nuclear energy and nuclear weapons issues, and she chaired the Science and Security Board of the Bulletin of Atomic Scientists, the group that sets the Bulletin’s famous “doomsday clock.” In 2006, MIT Press published a book she co-edited, Uncertainty Underground: Yucca Mountain and the Nation's High-Level Nuclear Waste. Dr. Macfarlane has published extensively in Science, Nature, Environmental Science and Technology, the Bulletin of the Atomic Scientists, and other journals. Dr. Macfarlane’s research has focused on technical, social, and policy aspects of nuclear energy production and nuclear waste management and disposal as well as regulation, nuclear nonproliferation, and energy policy.
Richard A. Meserve is Senior Of Counsel in the Washington, DC, office of Covington & Burling LLP, president emeritus of the Carnegie Institution for Science, and former chair of the US Nuclear Regulatory Commission. Early in his career, after obtaining a PhD in applied physics from Stanford and a JD from Harvard Law School, he served as law clerk to Supreme Court Justice Harry A. Blackmun and as legal counsel to the president’s science advisor. He has served on and chaired numerous legal and scientific committees, including many convened by the National Academies. Among other activities, he is the former president of the board of overseers of Harvard University and chair of the International Nuclear Safety Group (chartered by the International Atomic Energy Agency). He is a member of the National Academy of Engineering and served on its council. He is also a member of the American Academy of Arts and Sciences (former member of its council and trust), the American Philosophical Society, and the Council on Foreign Relations, a fellow the American Physical Society, and a foreign member of the Russian Academy of Sciences.
David Owens served as the Executive Vice President of Business Operations Group and Regulatory Affairs at Edison Electric Institute Inc. from October 1992 to June 2017. An executive with extensive experience in public policies concerning energy and utility operations, Mr. Owens is recognized as an industry expert on business transformation. After 36 years of service, he retired from the Edison Electric Institute, the association representing all U.S. Investor-owned electric companies. He was the first African-American to hold an officer title with the organization. He guided the association on issues affecting the future structure of the electric industry and the new rules in evolving competitive markets. He also spearheaded efforts to invest in the nation’s electric infrastructure with new technology enhancing energy efficiency with smart buildings, smart meters and smart grids. He served as Chief Engineer for the Division of Rates and Corporate Regulation with the Securities and Exchange Commission where he actively participated in landmark proceedings involving utility mergers, electric integration issues and utility financial disclosure. A driving force behind the founding of the American Association of Blacks in Energy, he has mentored generations of young men in careers in energy. He has been a Director of Xcel Energy Inc., since August 22, 2017. On December 21, 2018, he was nominated to be an independent director of the Puerto Rico Electric Power Authority. He now serves as vice chairman. He is a recognized expert in the energy field and has been a leader in shaping constructive public policy frameworks to support the industry’s transition to new and cleaner technologies. He served on Boards of the National Academy of Sciences and Chaired the National Institute of Standards and Technology Smart Grid Advisory Committee. He is a graduate of Howard University with a Bachelor and Masters of Engineering degrees, as well as a Masters in Engineering Administration from George Washington University.
James A. Rispoli , a licensed professional engineer in five states, is a Professor of Practice at the North Carolina State University. Additionally, he serves as a senior executive advisor for PT&C, LLC, an ENR-ranked engineering firm with over 30 years of experience serving owners in both operations and construction roles. Mr. Rispoli served as a career senior executive in the Department of Energy, and while Director, Office of Engineering and Construction Management, was invited by the Secretary to forego career status to accept a Presidential appointment. Thus, he served as an Assistant Secretary of Energy from 2005 through 2008, nominated by the President and unanimously confirmed by the United States Senate. Mr. Rispoli previously completed 27 years of service to the nation in the U.S. Navy, retiring as a Captain, Civil Engineer Corps. Subsequently, he was managing principal for an ENR top tier engineering firm and regional president of another top tier engineering firm in Hawaii, where his practice included multi-disciplinary engineering, environmental planning and engineering, and construction management. He left the private sector to join the Department of Energy’s new Office of Engineering and Construction Management in 1999. Articles and papers written by him on various subjects in engineering and leadership have been published, including seven published in either an edited book, or a refereed journal; and he has lectured extensively domestically and internationally in Japan, China, Singapore, Italy and the United Kingdom. Jim serves on the Board of the North Carolina Coastal Studies Institute, as well as on the Department of Energy’s Environmental Management Advisory Board. He earned his Bachelor of Engineering degree in Civil Engineering from Manhattan College, a Master of Science degree in Civil Engineering from the University of New Hampshire, and a Master’s degree in business from Central Michigan University. He is a Distinguished Member of the American Society of Civil Engineers (ASCE), a member of the National Academy of Construction (NAC), a Board Certified Environmental Engineer (National Academy of Environmental Engineers and Scientists), and a licensed professional engineer in five States. He is a member of two permanent boards/committees of the National Academies of Sciences, Engineering and Medicine (NASEM), and is chair of one of them. He also recently served on a study committee of the NASEM’s Nuclear and Radiation Studies Board. He is currently the chair of an ASCE committee charged with development of an ANSI standard on sustainable infrastructure. Mr. Rispoli has developed and teaches two graduate engineering courses at the North Carolina State University in Raleigh, NC, and initiated a curriculum for a graduate engineering concentration in facilities engineering which the College of Engineering is currently implementing.
Dr. Sola Talabi is a principal with Pittsburgh Technical, which is a nuclear consulting firm that specializes in advanced reactor design and deployment. He is also an adjunct professor of nuclear engineering at the University of Pittsburgh and the University of Michigan. Sola’s experience includes design, numerical computational analysis, manufacturing, installation and testing of nuclear power plant components. His experience includes serving as Risk Manager at Westinghouse Electric Company, where he was responsible for risk awareness, assessment and response for advanced reactors including AP1000 and SMR. He received the following degrees from Carnegie Mellon University: M.B.A., Ph.D. (Engineering and Public Policy) and M.Sc. (Mechanical Engineering). He received his B.Sc. in Engineering from University of Pittsburgh. Dr. Talabi is also a certified risk manager.
Dr. Steven Zinkle (NAE) is the Governor’s Chair Professor for Nuclear Materials in the Nuclear Engineering and Materials Science & Engineering Departments at the University of Tennessee at Knoxville. Prior to joining the faculty at the University of Tennessee, he was chief scientist for Oak Ridge National Laboratory’s (ORNL) Nuclear Science & Engineering Directorate and director of ORNL’s Materials Science and Technology Division. He also served as director of ORNL’s Metals and Ceramics Division, which merged into the Materials Science and Technology Division. Much of his research has utilized materials science to explore fundamental physical phenomena that are important for advanced nuclear energy applications, focusing on microstructure-property relationships. Dr. Zinkle’s research interests include the investigation of deformation and fracture mechanisms in structural materials, physical metallurgy of structural materials, and the investigation of radiation effects in ceramics, fuel systems and metallic alloys for fusion and fission energy systems. He has written over 300 peer-reviewed publications, and is a member of the Condensed Matter and Materials Research Committee. He is a fellow of 7 professional societies including the American Nuclear Society (ANS), TMS (The Minerals, Metals & Materials Society), ASM International, the Materials Research Society, and the American Physical Society. He received a B.S. and M.S. in nuclear engineering, an M.S. in materials science, and a Ph.D. in nuclear engineering, all from the University of Wisconsin at Madison.
The study committee was asked to identify opportunities and barriers to advanced nuclear reactor commercialization in the United States over the next 30 years as part of a decarbonization strategy. The report considers:
- Research, development, and demonstration
- Manufacturing, construction, and operational characteristics
- Economic, regulatory, societal, and business challenges
- Applications outside the electricity sector
- Role of the U.S. government
- National security and nonproliferation
- Future workforce and educational needs
Learn more about the study and read the official statement of task on the study website .
The funding for the report came from the Department of Energy and a philanthropic donation to the National Academy of Engineering by Dr. James J. Truchard.
There are many possible ways to achieve decarbonization, and there is a lot of uncertainty regarding what the future electricity system will look like in the United States as more low-carbon energy is deployed. This report doesn’t advocate for a specific path towards decarbonization, because the future marketplace will determine what technologies ultimately contribute to the future energy mix in the United States.
However, there is the potential for advanced nuclear technologies to play an important role in decarbonization, particularly if the cost barriers can be overcome. The committee sees the need to advance low-carbon technologies of all kinds to keep our options open and make sure we have tools that are available to confront this huge societal problem.
Nuclear waste is a serious problem that needs to be addressed. Nuclear fuel cycle issues were outside the scope of this report, but a recently published National Academies’ study covers that subject in detail. Merits and Viability of Different Nuclear Fuel Cycles and Technology Options and the Waste Aspects of Advanced Nuclear Reactors discusses nuclear fuel cycle options for both existing and advanced reactors, as well as nonproliferation and security considerations for these fuel cycles.
The committee met many times over the course of two years and held many information gathering sessions with briefings from federal agencies, vendors, and other stakeholders interested in advanced nuclear across a range of issues.
All recommendations in the report are consensus recommendations, agreed upon by the whole committee, comprised of experts from a wide array of backgrounds. The final report also went through rigorous peer review as part of the National Academies consensus study process.
One of the strengths of the National Academies is the tradition of bringing together recognized experts across many disciplines and facilitating collaboration. Careful steps are taken to convene diverse committees that have an appropriate range of expertise and represent a balance of perspectives. Stakeholders have the opportunity to nominate potential committee members at the beginning of the study, and all nominations are carefully considered. Committee members are always screened for possible conflicts of interest, and they serve as individual experts, not as representatives of organizations or interest groups. Learn more about the study committee for this report above.
The report has been presented to the study sponsors and congressional staff with interest in advanced nuclear technologies. Moving forward, additional dissemination briefings will be held for government, industry, scientific, and community stakeholders. If you are interested in requesting a briefing, please contact the study staff at [email protected] . Follow on events, including a workshop, are planned for late 2023.

Download the Report
- Read Online
- Download PDF
- Report Findings and Recommendations
- Report Highlights
- Public Briefing Video
- Public Briefing Slides
- Press Release

RELATED RESOURCES
This study was organized by the National Academies’ Board on Energy and Environmental Systems and the National Academy of Engineering.
- Future Energy Challenges
- Advanced Nuclear Reactors
- Reference Manager
- Simple TEXT file
People also looked at
Editorial article, editorial: experimental and simulation research on nuclear reactor thermal-hydraulics.
- 1 Department of Nuclear Engineering and Technology, School of Energy and Power Engineering, Chongqing University, Chongqing, China
- 2 Department of Engineering Physics, University of Wisconsin-Madison, Madison, WI, United States
- 3 Helmholtz-Zentrum Dresden-Rossendorf, Dresden, Germany
Editorial on the Research Topic Experimental and simulation research on nuclear reactor thermal-hydraulics
The researches on nuclear reactor thermal-hydraulics have achieved outstanding progresses in the past decades. In recent years, basic research on multiphase flow dynamics and corresponding measurement technology, as well as preliminary research on Gen IV reactors based on experiments and simulations are attracting more and more attention. However, the inside complicated physics and outside extreme conditions will also bring risks and challenges to the development of nuclear industry.
Prof. Liangming Pan from Chongqing University held the first annual academic meeting of the branch of Nuclear Reactor Thermal-Hydraulics and Fluid Mechanics of Chinese Nuclear Society in October 2021. Several high-quality papers were selected from this conference. Therefore, Prof. Pan proposed the Research Topic “ Experimental and Simulation Research on Nuclear Reactor Thermal-Hydraulics ” in the journal Frontiers in Energy Research . This research topic aims to promote the novel experimental and numerical investigations on relevant issues refer to nuclear reactor thermal-hydraulics, which is of great significance to the system optimization and safety evaluation of nuclear power plants. Finally, 14 articles were collected and published in this topic, covering the experimental and numerical research of thermal hydraulic problems in light water reactors to supercritical coolant reactors.
Most papers are relevant to the thermal hydraulic problems in light water reactors. Li et al. have contributed an article: “ Research on Countercurrent Flow Limitation in Reactor Hot Leg at the Loss of Coolant Accident-Thermal hydraulic Calculation with System Code RELAP5 ”. Meng et al. have presented an article entitled: “ Research of Thermal Hydraulic Conditions Effect on PWR CIPS Risk ”. Yu et al. have presented an article: “ An Innovative Investigation on Fluid-to-Fluid Modeling of Post-Dryout Heat Transfer in Thermal Energy Systems ”. Zhu et al. have contributed an article: “ Comparison of Intergroup Mass Transfer Coefficient Correlations in Two-Group IATE for Subcooled Boiling Flow ”. Ding et al. have contributed their research relevant to: “ Experimental Research of Flow Distribution at Reactor Core Inlet of ACP100 ”. Li et al. have presented article in title of “ Experimental Study on Stratification Morphology of the Molten Pool during Severe Accident ”.
Several research papers are about the supercritical coolant. Zhang et al. have contributed an article: “ Experimental Study on Prototype of Printed Circuit Heat Exchanger ”. Zhu et al. have presented an article entitled: “ Dynamic Characteristic Study of Supercritical CO 2 Recompression Brayton Cycle System ”. Min et al. have presented their research article of: “ Study of Supercritical CO 2 Physical Property Calculation Models ”.
Several articles are related to the high temperature gas-cooled reactor. Qin et al. have presented an article entitled: “ Numerical Investigation of Hot Helium Flow Homogenizer on Inter-Unit Flow Rate Uniformity of HTGR Once through Steam Generator ”. Qu et al. have contributed an article: “ Characteristics Analysis of Combined Cycle Coupled With High Temperature Gas-Cooled Reactor Based on Progressive Optimization ”.
Meanwhile, there are several articles about other issues. Wang et al. have contributed the research paper of: “ Neutronics and Thermal Hydraulics Coupling Analysis on Novel Organic Cooled Reactor Based on Single-Channel Model ”. Fang et al. have contributed an article: “ Numerical Study on Heat Transfer and Enhancement Mechanism in PCM-Filled Shell-and-Tube Heat Exchangers ”. Su et al. have contributed an article entitled: “ Development and Assessment of an Isotropic Four-Equation Model for Heat Transfer of Low Prandtl Number Fluids ”.
Through revision and update for almost 1 year, this topic finally closed with above 14 papers published in the journal Frontiers in Energy Research. This topic presents a chance to public to follow these high-quality papers selected from this conference. The journal Frontiers in Energy Research will always open to accept more papers from international conferences. All the editors are welcomed to contact the journal for further information.
Author contributions
LZ is the leading author. LP is the corresponding author. JW and WD contribute the review. All authors approved the submitted version.
Conflict of interest
The authors declare that the research was conducted in the absence of any commercial or financial relationships that could be construed as a potential conflict of interest.
Publisher’s note
All claims expressed in this article are solely those of the authors and do not necessarily represent those of their affiliated organizations, or those of the publisher, the editors and the reviewers. Any product that may be evaluated in this article, or claim that may be made by its manufacturer, is not guaranteed or endorsed by the publisher.
Keywords: nuclear reactor, thermal, hydraulics, experiments, simulation
Citation: Zhang L, Pan L, Wang J and Ding W (2022) Editorial: Experimental and simulation research on nuclear reactor thermal-hydraulics. Front. Energy Res. 10:1028698. doi: 10.3389/fenrg.2022.1028698
Received: 26 August 2022; Accepted: 06 September 2022; Published: 23 September 2022.
Edited and reviewed by:
Copyright © 2022 Zhang, Pan, Wang and Ding. This is an open-access article distributed under the terms of the Creative Commons Attribution License (CC BY). The use, distribution or reproduction in other forums is permitted, provided the original author(s) and the copyright owner(s) are credited and that the original publication in this journal is cited, in accordance with accepted academic practice. No use, distribution or reproduction is permitted which does not comply with these terms.
*Correspondence: Luteng Zhang, [email protected] ; Liangming Pan, [email protected]
This article is part of the Research Topic
Experimental and Simulation Research on Nuclear Reactor Thermal-Hydraulics
Research Reactors
- Many of the world's nuclear reactors are used for research and training, materials testing, or the production of radioisotopes for medicine and industry. They are basically neutron factories.
- These are much smaller than power reactors or those propelling ships, and many are on university campuses.
- There are about 220 such reactors operating, in 53 countries.
- Some operate with high-enriched uranium fuel, and international efforts have substituted high-assay low-enriched fuel in most of these. Some radioisotope production also uses high-enriched uranium as target material for neutrons, and this is being phased out in favour of low-enriched uranium.
Research reactors comprise a wide range of civil and commercial nuclear reactors which are generally not used for power generation. The term is used here to include test reactors, which are more powerful than most. The primary purpose of research reactors is to provide a neutron source for research and other purposes. Their output (neutron beams) can have different characteristics depending on use. They are small relative to power reactors whose primary function is to produce heat to make useful amounts of electricity. They are essentially net energy users. Their power is designated in megawatts (or kilowatts) thermal (MWth or MWt), but here we will use simply MW (or kW). Most range up to 100 MW, compared with 3000 MW ( i.e. 1000 MWe) for a typical power reactor. In fact the total power of the world's 250 research reactors is little over 3000 MW.
Research reactors are simpler than power reactors and operate at lower temperatures. They need far less fuel, and far less fission products build up as the fuel is used. On the other hand, their fuel requires uranium that is more highly enriched, typically up to 20% U-235, known as high-assay low-enriched uranium (HALEU), although some older ones still use 93% U-235. They also have a very high power density in the core, which requires special design features. Like power reactors, the core needs cooling, usually passively and only the higher-powered test reactors need forced cooling. Usually a moderator is required to slow down the neutrons and enhance fission. As neutron production is their main function, most research reactors also need a reflector to reduce neutron loss from the core.
Operational research reactors
Nearly all of the world’s research reactors operate with thermal (slow) neutrons; Russia claims that its BOR-60 at Dimitrovgrad is the only fast neutron research reactor.* It started up in 1969 and is to be replaced after the end of 2020 with the MBIR, with four times the irradiation capacity. There is a world shortage of fast reactor research capacity, especially for fast neutron materials testing for Generation IV reactor developments. In February 2018 a bipartisan bill passed by the US House of Representatives authorized $2 billion for the construction of a "versatile reactor-based fast neutron source, which shall operate as a national user facility" by 2026. It will be a research reactor for "development of advance reactor designs, materials and nuclear fuels" of at least 300 MWt.
* Several small experimental reactors – CEFR, FBTR, Joyo – fall into the broad category of research reactors in that they are not designed to produce power for the grid, but they do not generally operate as neutron irradiation and research facilities for third parties (although CEFR may do so to some extent).
As of June 2021 the IAEA research reactor database showed that there were 223 operational research reactors, 11 under construction, 16 planned, 27 temporarily or in extended shutdown, 58 permanently shutdown, and 511 decommissioned or undergoing decommissioning. A majority of the operational and temporary shutdown research reactors are over 40 years old.
Many research reactors are used with international collaboration, and the products of those used for isotope production are traded internationally. The IAEA has designated two international research hubs based on research reactors, giving them ICERR (International Centres based on Research Reactors) status valid for five years. The first is in France, based on CEA’s Saclay and Cadrache facilities, the second is in Russia, the Research Institute of Atomic Reactors (RIAR) at Dimitrovgrad, with six research reactors available to IAEA member states.
Types of research reactor
There is a much wider array of designs in use for research reactors than for power reactors, where 80% of the world's plants are of just two similar types. They also have different operating modes, producing energy which may be steady or pulsed.
A common design (47 units) is the pool-type reactor, where the core is a cluster of fuel elements sitting in a large pool of water. Among the fuel elements are control rods and empty channels for experimental materials. Each element comprises several (e.g. 18) curved aluminium-clad fuel plates in a vertical box. The water both moderates and cools the reactor, and graphite or beryllium is generally used for the reflector, although other materials may also be used. Apertures to access the neutron beams are set in the wall of the pool. Tank type research reactors (21 units) are similar, except that cooling is more active.
The TRIGA (Training, Research, Isotopes, General Atomics) reactor is a common pool-type design (36 units) with three generations of design commissioned since 1960. The core consists of 60-100 cylindrical fuel elements about 37 mm in diameter, 722 mm long with aluminium or steel cladding enclosing self-moderating uranium zirconium hydride fuel. Enrichment today is HALEU - under 20%. The core sits in a pool of water and generally uses graphite as a reflector. This kind of reactor can safely be pulsed to very high power levels (up to 22,000 MW) for fractions of a second. Its UZrH fuel has a very strong prompt negative temperature coefficient, and a rapid increase in power is quickly cut short by the negative reactivity effect of the fuel.
Other designs are moderated by heavy water (c. 10 units) or graphite. A few are fast reactors, which require no moderator and can use a mixture of uranium and plutonium as fuel. Homogenous type reactors have a core comprising a solution of uranium salts as a liquid, contained in a tank about 300 mm diameter. The simple design made them popular early on, but only a few are now operating, and all except two of those (20 kW) are very low power.
Research reactors have a wide range of uses, including analysis and testing of materials, and production of radioisotopes. Their capabilities are applied in many fields, within the nuclear industry as well as in fusion research, environmental science, advanced materials development, drug design and nuclear medicine.
Four high-flux research reactors are in operation: the 45 MW High Flux Reactor in Petten (Netherlands), which is nearing the end of its design life, the upgraded 85 MW High Flux Isotope Reactor at Oak Ridge National Laboratory (USA), the 20 MW Heinz Maier-Leibnitz FRM II (Germany) and the 100 MW PIK high-flux reactor at St Petersburg (Russia).
Most research reactors (over 160) are principally for research, although some may also produce radioisotopes. As expensive scientific facilities, they tend to be multi-purpose, and many have been operating for more than 30 years.
About 820 research and test reactors have been built worldwide, 307 of these in the USA and 121 in Russia.
In 2020, Russia has most operational research reactors, followed by the USA (see above table ). Many research reactors were built in the 1960s and 1970s. The peak number operating was in 1975, with 373 in 55 countries.
Neutron beams are uniquely suited to studying the structure and dynamics of materials at the atomic level. Neutron scattering is used to examine samples under different conditions such as variations in vacuum pressure, high temperature, low temperature and magnetic field, essentially under real-world conditions.
Using neutron activation analysis, it is possible to measure minute quantities of an element. Atoms in a sample are made radioactive by exposure to neutrons in a reactor. The characteristic radiation each element emits can then be detected.
Neutron activation is the only common way that a stable material can be made radioactive. It is used to produce the radioisotopes, widely used in industry and medicine, by bombarding particular elements with neutrons so that the target nucleus has a neutron added. For example, yttrium-90 microspheres to treat liver cancer are produced by bombarding yttrium-89 with neutrons.
Neutron activation can result in fission. The most widely used isotope in nuclear medicine is technetium-99m, a decay product of molybdenum-99*. This is usually produced by irradiating a target of uranium foil with neutrons (for a week or so) and then separating the molybdenum-99 from the other fission products of U-235 in a hot cell – the Mo-99 being about 6% of the fission products. Most Mo-99/Tc-99m production has been using HEU targets, but increasingly LEU is favoured and HEU is being phased out. (Mo-99 may also be produced in power reactors by neutron activation/capture in Mo-98 targets.)
* Technetium generators, a lead pot enclosing a glass tube containing the radioisotope, are supplied to hospitals from the nuclear reactor where the isotopes are made. They contain molybdenum-99, with a half-life of 66 hours, which progressively decays to technetium-99m, with a half-life of 6 hours. The Tc-99 is washed out of the lead pot by saline solution when it is required. It is then attached to a particular protein for administering to the patient. After two weeks or less the generator is returned for recharging, since it loses 22% of its product every 24 hours.
Research reactors can also be used for industrial processing. Neutron transmutation doping (NTD) changes the properties of silicon, making it highly conductive of electricity. Large, single crystals of silicon shaped into ingots, are irradiated inside a reactor reflector vessel. Here the neutrons change one atom of silicon in every billion to phosphorus. The irradiated silicon is sliced into chips and used for a wide variety of advanced computer applications. NTD increases the efficiency of the silicon in conducting electricity, an essential characteristic for the electronics industry.
In materials testing reactors (MTRs), materials are also subject to intense neutron irradiation to study changes. For instance, some steels become brittle, and alloys which resist embrittlement must be used in nuclear reactors. The international project to build the 100 MWt Jules Horowitz reactor at Cadrache in France will enable research on materials which will be vital in Generation IV nuclear plants. It is designed to produce very high neutron flux – about twice that of France’s Osiris MTR. Civil works were more than 80% complete at the end of 2014 and test loops are being fitted out. It is due to be operational in 2021. At present Belgium’s BR2 is the largest MTR in Europe.
A very small US reactor is designed to perform research and development on various operational features of microreactors to improve their integration with end-user applications. The Department of Energy plans to build the Microreactor Applications Research Validation and Evaluation (MARVEL) reactor of 100 kWt at Idaho National Laboratory’s Transient Reactor Test Facility (TREAT). It will be based on the 1965 SNAP-10A – the only US fission reactor to have been launched into space. Fuel will be TRIGA HALEU UZrH, cooling will be by sodium or salt for operating temperature of 500-550°C. Power conversion will be by Stirling engines. This will be the first US small-scale reactor for such R&D purposes in 40 years. The DOE Microreactor Program is focused on very small, factory fabricated, transportable reactors to provide power and heat for decentralized generation in civilian, industrial and defence energy sectors.
Like power reactors, research reactors are covered by IAEA safety inspections and safeguards, because of their potential for making nuclear weapons. India's 1974 explosion was the result of plutonium production in a large, but internationally unsupervised, research reactor which closed at the end of 2010.
One of the more interesting and powerful test reactors was Plum Brook in Ohio, USA, which operated for NASA over 1961-73 and was designed to research nuclear power for aircraft, then nuclear-powered rockets and spacecraft. It was 60 MW pool-type, light water cooled and moderated, with a very high neutron flux – 420 trillion/cm 2 /s.
See also paper on Australian Research Reactors .
Research reactor fuel is more highly enriched (typically about 20% today) than power reactor fuel. It is high-assay low-enriched uranium (HALEU), which means it has less U-238, hence the used fuel has fewer actinides and less heat from radioactive decay. The proportion of fission products is not much different from used power reactor fuel.
Fuel assemblies are typically plates or cylinders of uranium-aluminium alloy (U-Al) clad with pure aluminium. They are different from the ceramic UO 2 pellets enclosed in Zircaloy cladding used in power reactors. Only a few kilograms of uranium is needed to fuel a research reactor, albeit more highly enriched, compared with perhaps a hundred tonnes in a power reactor. Research reactors typically operate at low temperatures (coolant below 100ºC), but the operating conditions are severe in other ways. While power reactor fuel operates at power density of about 5 kW/cc, a research reactor fuel may be at 17 kW/cc in the fuel meat. Also burnup is very much higher, so the fuel must withstand structural damage from fission and accommodate more fission products.
Five Russian-designed research reactors in Russia use high-enriched uranyl sulfate liquid fuel. One in Uzbekistan was decommissioned in 2014 and the fuel airlifted to Mayak.
Highly-enriched uranium (HEU – >20% U-235) allowed more compact cores, with high neutron fluxes and also longer times between refuelling. Therefore many reactors up to the late 1970s used it, and most state-of-the-art reactors had 93% enriched fuel. A total of 171 were built with a HEU core, and by 2020, 71 of these had been converted to use LEU and 28 had been shut down. The IAEA said "work remains to be done" on 72 still using HEU.
Since the early 1970s security concerns have grown, especially since many research reactors are located at universities and other civilian locations with much lower security than military weapons establishments where much larger quantities of HEU exist. Since 1978 only one reactor, the FRM-II at Garching in Germany, has been built with HEU fuel, while more than 20 have been commissioned on LEU fuel in 16 countries. The Jules Horowitz reactor in France will start up on uranium silicide fuel enriched to 27%, since the planned high-density U-Mo fuel will not be ready in time for it.)
The question of enrichment was a major focus of the UN-sponsored International Nuclear Fuel Cycle Evaluation in 1980. It concluded that to guard against weapons proliferation from the HEU fuels then commonly used in research reactors, enrichment should be reduced to no more than 20% U-235, i.e. HALEU. This followed a similar initiative by the USA in 1978 when its programme for Reduced Enrichment for Research and Test Reactors (RERTR) was launched.
Most research reactors using HEU fuel were supplied by the USA and Russia, hence efforts to deal with the problem are largely their initiative. The RERTR programme concentrates on reactors over 1 MW which have significant fuel requirements. However, some are defence-related (mostly in Russia) or impractical for other reasons. Some have lifetime cores which require no refuelling, so there is little incentive to convert them. In February 2020 the IAEA announced that almost 3500 kg of HEU fuel had been removed from research reactor sites around the world.
In 2004 the US National Nuclear Security Administration (NNSA) set up the Global Threat Reduction Initiative (GTRI), which is congruent with RERTR objectives though it is mainly tackling the disposition of HEU fuel (fresh and used) and other radiological materials. RERTR is now a major part of GTRI. GTRI claims accelerated removal of Russian-origin fresh and used HEU fuel to Russia and of US-origin fuel to the USA.
In particular, to January 2010, 915 kg of fresh and used HEU fuel had been returned to Russia from at least 11 countries including Hungary (155 kg), Serbia, Romania, Libya, Uzbekistan, Poland, Czech Republic, Latvia and Vietnam. In 2016, the last of 700 kg of HEU fuel from Poland joined them after Poland’s 30 MWt Maria reactor was fully converted to HALEU in 2014. And to January 2010, 1240 kg of US-origin HEU fuel had been returned from Europe, Israel, Turkey, Latin America, Japan and Southeast Asia. Since then more has come from some of these countries and from Belgium, Italy, Chile, Mexico, Ukraine, South Africa and Austria. In mid-2016 several hundred kilograms more was returned from Japan’s Fast Critical Assembly, along with some plutonium. The HEU returned to the USA is downblended, with the plutonium disposed of at the Waste Isolation Pilot Plant (WIPP) in New Mexico. In 2011, 33kg of HEU in Kazakhstan was downblended to LEU there and returned to the Institute of Nuclear Physics for use once the WWR-K reactor was converted to use it. Over 2015-19 more than 200 kg of HEU from two research reactors at Canada's Chalk River, Ontario, was moved to Savannah River, South Carolina.
After a hiatus of six years the US government late in 2008 had converted five university research reactors from using high- to low-enriched uranium fuel.* It was reported in 2006 that worldwide, 40 remained to be converted under the RERTR scheme using currently-available fuels, and 19 more await development of high-density fuel. At the end of 2017 these included the Advanced Test Reactor and the Advanced Test Reactor Critical Assembly, both at Idaho National Laboratory; the High Flux Isotope Reactor at Oak Ridge National Laboratory, Tennessee; the Massachusetts Institute of Technology Reactor (MITR) in Cambridge, Massachusetts; the National Bureau of Standards Reactor in Gaithersburg, Maryland; and the University of Missouri Research Reactor (MURR) in Columbia, Missouri.
* Texas A&M, University of Florida, Purdue, Oregon State and Washington State University reactors can now operate on fuel of less than 20% enrichment, and the University of Wisconsin reactor was to be converted in 2009.
China converted the first of its miniature neutron source reactors (MNSRs) to a denser LEU fuel in 2016, in partnership with the US NNSA and Argonne National Laboratory. Other MNSRs are in China, Ghana, Nigeria, Iran, Pakistan and Syria. The MNSR core has about one kilogram of of 90% enriched fuel. HEU from Ghana and Nigeria at least has been returned to the China Institute of Atomic Energy.
By December 2018 NNSA’s Material Management and Minimization (M3) Reactor Conversion Program had converted or verified shutdown of more than 90 HEU-fuelled research and test reactors worldwide over the past 30 years, and had confirmed the disposition of more than 6.7 tonnes of HEU and plutonium from 33 countries.
New LEU fuels
These RERTR programmes have led to the development and qualification of new, high density, high-assay low enriched uranium (HALEU) fuels. The original fuel density was about 1.3-1.7 g/cm 3 uranium. Lowering the enrichment meant that the density had to be increased. Initially this was to 2.3-3.2 g/cm 3 with existing U-Al fuel types.
To September 2009, 67 research reactors (17 in USA) had been converted to low-enriched uranium silicide fuel or shut down, including major reactors in Ukraine, Uzbekistan and South Africa. Another 34 are convertible using present fuels. A further 28, mostly Russian designs but including two US university reactors, need higher-density fuels not yet available. The goal was to convert or shut 129 reactors by 2018. US exports of HEU declined from 700 kg/yr in mid 1970s to almost zero by 1993.
The Soviet Union made similar efforts from 1978, and produced fuel of 2.5 g/cm3 with enrichment reduced from 90 to 36%. It largely stopped exports of 90% enriched fuel in the 1980s. No Russian research reactor has yet been converted to LEU, and the Russian effort has been focused on its reactors in other countries. However, Russia has been looking at the feasibility of converting six domestic reactors,* while others will require high-density fuels. Early in 2012 a joint Rosatom-US NNSA project completed studies on converting two of these six reactors to HALEU fuel. Another 68 Russian reactors fall outside the scope of the conversion program because they are defence-related or special purpose. A December 2010 agreement with the US DOE relates to feasibility studies on converting six Russian research reactors to HALEU.
* IR-8, OR, and Argus at Kurchatov Institute, IRT-MEPHI at Moscow Engineering Physics Inst, IRT-T at Tomsk Polytechnic Inst, MIR at Dimitrovgrad Research Inst.
The first generation of new HALEU fuels used uranium and silicon (U 3 Si 2 -Al – uranium silicide dispersed in aluminium) – at 4.8 g/cm 3 . There have been successful tests with denser U 3 Si-Al fuel plates up to 6.1 g/cm 3 , but US development of these silicide fuels ceased in 1989 and did not recommence until 1996. In Russia the Bochvar Institute is developing fabrication technology for uranium disilicide pellets as part of developing accident-tolerant fuels for power reactors. As well as high uranium density, it points to high thermal conductivity and low heat capacity of silicide fuel. The presence of silicon makes reprocessing more difficult.
An international effort is underway to develop, qualify and license a high-density fuel based on U-Mo alloy dispersed in aluminium, with a density of 6-8g/cm 3 . The principal organizations involved are the US RERTR program at the Idaho National laboratory (INL) since 1996, the French U-Mo Group (CEA, CERCA, COGEMA, Framatome-ANP and Technicatome) since 1999 and the Argentine Atomic Energy Commission (CNEA) since 2000. This development work was undertaken to provide fuels which can extend the use of LEU to those reactors requiring higher densities than available in silicide dispersions and to provide a fuel that can be more easily reprocessed than the silicide type. Approval of this fuel was expected in 2006 but tests since 2003 failed to confirm performance due to unstable swelling under high irradiation. Attention has turned to monolithic U-Mo fuel.
In 2012, Belgium, France, South Korea and the USA agreed to cooperate in the development of high-density HALEU fuel production technology using centrifugal atomization technology developed by the Korea Atomic Energy Research Institute (KAERI). The USA provided 110 kg of HALEU in June 2013 for KAERI to manufacture 100 kg of atomized U-Mo powder. In January 2014, the powder was shipped to France for fabrication into fuel elements by Areva's research reactor fuel manufacturer CERCA. Testing of this monolithiic HALEU-Mo fuel began in the Advanced Test Reactor at Idaho National Laboratory (INL) in October 2015, and in April 2017 KAERI announced the successful completion of those verification tests.
This monolithic HALEU-Mo fuel is essentially pure metal with 10% Mo, instead of a dispersion of U-Mo in aluminium. The uranium density is 15.6 g/cm 3 and this would enable every research reactor in the world to convert from HEU to HALEU fuel without loss of performance. In December 2017 the NNSA presented a preliminary report of HALEU-Mo monolithic fuel to the Nuclear Regulatory Commission as a first step in qualifying it, and being able to convert the six remaining US high-performance research reactors from HEU fuel. In February 2020 NNSA awarded a contract to BWX Technologies to prepare for HALEU-Mo fuel production at Lynchburg, Virginia.
KAERI said the results from the testing of the fuel will be used to obtain a construction licence for a new research reactor planned in Busan, which it hopes will be the first application for this HALEU-Mo alloy fuel.
In Russia, a parallel Russian RERTR programme funded jointly by Rosatom and the US RERTR programme has been working since 1999 to develop U-Mo dispersion fuel with a density of 2-6 g/cm 3 for use in Russian-designed research and test reactors. However, this has not fulfilled expectations.
All solid fuel is aluminium-clad.
Used fuel from research reactors usually generates less than 2kW/m 3 of decay heat, so is classed as intermediate-level waste (ILW), though the activity may still be quite high.
U-Al fuels can be reprocessed by Areva in France, and U-Mo fuels may also be reprocessed there. U-Si and TRIGA fuels are not readily reprocessed in conventional facilities. However, at least one commercial operator has confirmed that U-Si fuels may be reprocessed in existing plants if diluted with appropriate quantities of other fuels, such as U-Al.
To answer concerns about interim storage of spent research fuel around the world, the USA launched a program to take back US-origin spent fuel for disposal and nearly half a tonne of U-235 from such HEU fuel has been returned. By the time the program was to end with fuel discharged in 2006, U-Mo fuel was expected to be available. Due to the slippage in target date, the US take-back program has now been extended by ten years.
Disposal of high-enriched or even 20% enriched fuel needs to address problems of criticality and requires the use of neutron absorbers or diluting or spreading it out in some way.
In Russia, a parallel trilateral program involving IAEA and the USA to move 2 tonnes of HEU and 2.5 tonnes of LEU spent fuel to the Mayak reprocessing complex near Chelyabinsk over the ten years to 2012. This Russian Research Reactor Fuel Return Program (RRR FRT) envisaged 38 shipments (of both fresh and used fuel) from ten countries over 2005-08, then 8+ shipments from six countries to remove all HEU fuel discharged before reactors converted to HALEU or shut down. Seventeen countries have Soviet-supplied research reactors, and there are 25 such reactors outside Russia, 15 of them still operational. Since Libya joined the program in 2004, only North Korea objects to it.
The 2004 joint US-Russian program to retrieve used fuel from 14 countries (Belarus, Bulgaria, Hungary, Vietnam, Germany, Kazakhstan, Latvia, Libya, Poland, Romania, Serbia, Uzbekistan, Ukraine and the Czech Republic) has been extended from 2016 to 2024.
Aqueous homogeneous reactors
Aqueous homogeneous reactors (AHRs) have the fuel mixed with the moderator as a liquid. Typically, low-enriched uranium nitrate is in aqueous solution. About 30 AHRs have been built as research reactors and have the advantage of being self-regulating and having the fission products continuously removed from the circulating fuel. However, corrosion problems and the propensity of water to decompose radiolytically (due to fission fragments) releasing gas bubbles have been design problems.
A series of three reactors were built at Los Alamos National Laboratory in the mid-19402/early 1950s. The first AHR at Oak Ridge National Laboratory went critical in 1952, and attained full power of one megawatt in 1953. A second one there reached 5 MW in 1958. Plans for a 70 to 150 MWe commercial unit did not proceed. A 1 MWt AHR operated in the Netherlands 1974-77 using Th-HEU MOX fuel. At Russia's Kurchatov Institute the 20 kW ARGUS AHR has operated since 1981, and R&D on producing Sr-89 and Mo-99 from it is ongoing. The Mo-99 is extremely pure, making the design potentially valuable for its commercial production. As of 2006, only five AHRs were operating, but the concept of extracting medical isotopes directly from the fuel has sparked renewed interest in them. The USA, China and Russia are assessing the prospects of using AHRs for commercial radioisotope production.
In 2008, the IAEA summarized: "The use of solution reactors for the production of medical isotopes is potentially advantageous because of their low cost, small critical mass, inherent passive safety, and simplified fuel handling, processing and purification characteristics. These advantages stem partly from the fluid nature of the fuel and partly from the homogeneous mixture of the fuel and moderator in that an aqueous homogeneous reactor combines the attributes of liquid fuel homogeneous reactors with those of water moderated heterogeneous reactors. If practical methods for handling a radioactive aqueous fuel system are implemented, the inherent simplicity of this type of reactor should result in considerable economic gains in the production of medical isotopes." Thermal power can be 50-300 MW at low temperature and pressure, and low enriched uranium fuel used. However, recovering desired isotopes on a continuous production basis remains to be demonstrated. As well as those in solution, a number of volatile radioisotopes used in nuclear medicine can be recovered from the off-gas arising from radiolytic 'boiling'. For isotopes such a Sr-89 this is very much more efficient than alternative production methods.
At the end of 2007, Babcock & Wilcox (B&W) notified the US Nuclear Regulatory Commission that it intended to apply for a licence to construct and operate a Medical Isotope Production System (MIPS) – an AHR system with low-enriched uranium in small 100-200 kW units for Mo-99 production. A single production facility could have four such reactors. B&W expects a five-year lead time to first production. The fuel is brought to criticality in a 200-litre vessel. As fission proceeds, the solution is circulated through an extraction facility to remove the Mo-99 and then back into the reactor vessel, which is at low temperature and pressure. In January 2009, B&W Technical Services Group signed an agreement with radiopharmaceutical and medical device supplier Covidien to develop technology for the MIPS. In October 2012 Covidien pulled out of the joint venture with B&W “after learning that the time and cost involved with the project would be greater than originally expected.” B&W appears to have dropped the MIPS.
Research reactors originally with high-enriched uranium (HEU) fuel
Data from IAEA: Nuclear Research Reactors in the World, 2000, plus Nigeria. NB some now are converted to LEU or closed. MNSR = miniature neutron source reactor, Chinese copy of Slowpoke. crit fast = very low power critical assembly for fast neutrons.
Notes & References
General sources.
IAEA, Research Reactors database IAEA, Nuclear Research Reactors in the World, reference data series #3, Sept 2000 Research Reactors: an overview, by Colin West, ANS Nuclear News, Oct 1997 IAEA, Research Reactor Facility Characteristics, 1985 Research reactors under threat, by W.Krull, Nucl.Eng.Intl. Oct 2000 O.Bukharin, 2002, Making fuel less tempting, Bull. Atomic Scientists, July-Aug 2002 Travelli, A 2002, Progress of the RERTR program in 2001 Reduced Enrichment for Research and Test Reactors (RERTR) Program website Travelli, A 2002, Status and Progress of the RERTR Program in the Year 2002, RERTR conference November 2002 Snelgrove JL 2003, Qualification and Licensing of U-Mo Fuel, RRFM conference, March 2003 NuclearFuel 17/3/03, 22/11/04, 26/3/07, 20/10/08 Wachs, Daniel, 2010, Reactor Conversion, Nuclear Engineering International January 2010 National Nuclear Security Administration website Mark D. Bowles and Robert S. Arrighi, NASA's Nuclear Frontier – The Plum Brook Reactor Facility, 1941-2002, Monographs in Aerospace History Series No. 33, SP-2004-4533, NASA History Division, August 2004 Homogeneous Aqueous Solution Nuclear Reactors for the Production of Mo-99 and other Short Lived Radioisotopes, International Atomic Energy Agency, IAEA-TECDOC-1601, ISBN 9789201097088 (September 2008) B&W and Covidien to Develop U.S. Source of Key Medical Isotope (26 Jan 2009) National Nuclear Security Administration, NNSA reaches milestone in developing new nuclear fuel for U.S. high-performance research reactors (20 December 2017)
Related information
Suggestions or feedback?
MIT News | Massachusetts Institute of Technology
- Machine learning
- Social justice
- Black holes
- Classes and programs
Departments
- Aeronautics and Astronautics
- Brain and Cognitive Sciences
- Architecture
- Political Science
- Mechanical Engineering
Centers, Labs, & Programs
- Abdul Latif Jameel Poverty Action Lab (J-PAL)
- Picower Institute for Learning and Memory
- Lincoln Laboratory
- School of Architecture + Planning
- School of Engineering
- School of Humanities, Arts, and Social Sciences
- Sloan School of Management
- School of Science
- MIT Schwarzman College of Computing
Professor Emeritus David Lanning, nuclear engineer and key contributor to the MIT Reactor, dies at 96
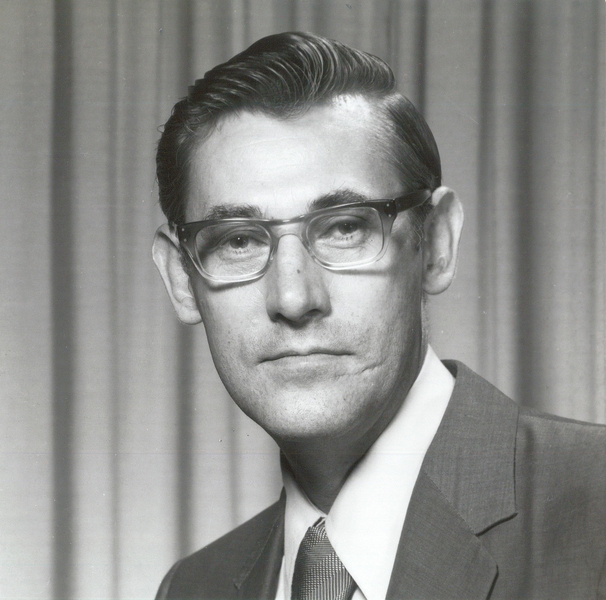
Previous image Next image
David Lanning, MIT professor emeritus of nuclear science and engineering and a key contributor to the MIT Reactor project, passed away on April 26 at the Lahey Clinic in Burlington, Massachusetts, at the age of 96.
Born in Baker, Oregon, on March 30, 1928, Lanning graduated in 1951 from the University of Oregon with a BS in physics. While taking night classes in nuclear engineering, in lieu of an available degree program at the time, he started his career path working for General Electric in Richland, Washington. There he conducted critical-mass studies for handling and designing safe plutonium-bearing systems in separation plants at the Hanford Atomic Products Operation, making him a pioneer in nuclear fuel cycle management.
Lanning was then involved in the design, construction, and startup of the Physical Constants Testing Reactor (PCTR). As one of the few people qualified to operate the experimental reactor, he trained others to safely assess and handle its highly radioactive components.
Lanning supervised experiments at the PCTR to find the critical conditions of various lattices in a safe manner and conduct reactivity measurements to determine relative flux distributions. This primed him to be an indispensable asset to the MIT Reactor (MITR), which was being constructed on the opposite side of the country.
An early authority in nuclear engineering comes to MIT
Lanning came to MIT in 1957 to join what was being called the “MIT Reactor Project” after being recruited by the MITR’s designer and first director, Theos “Tommy” J. Thompson, to serve as one of the MITR’s first operating supervisors. With only a handful of people on the operations team at the time, Lanning also completed the emergency plan and startup procedures for the MITR, which achieved criticality on July 21, 1958.
In addition to becoming a faculty member in the Department of Nuclear Engineering in 1962, Lanning’s roles at the MITR went from reactor operations superintendent in the 1950s and early 1960s, to assistant director in 1962, and then acting director in 1963, when Thompson went on sabbatical.
In his faculty position, Lanning took responsibility for supervising lab subjects and research projects at the MITR, including the Heavy Water Lattice Project. This project supported the thesis work of more than 30 students doing experimental studies of sub-critical uranium fuel rods — including Lanning’s own thesis. He received his PhD in nuclear engineering from MIT in fall 1963.
Lanning decided to leave MIT in July 1965 and return to Hanford as the manager of their Reactor Neutronics Section. Despite not having plans to return to work for MIT, Lanning agreed when Thompson requested that he renew his MITR operator’s license shortly after leaving.
“Because of his thorough familiarity with our facility, it is anticipated that Dr. Lanning may be asked to return to MIT for temporary tours of duty at our reactor. It is always possible that there may be changes in the key personnel presently operating the MIT Reactor and the possible availability of Dr. Lanning to fill in, even temporarily, could be a very important factor in maintaining a high level of competence at the reactor during its continued operation,” Theos J. Thompson wrote in a letter to the Atomic Energy Commission on Sept. 21, 1965
One modification, many changes
This was an invaluable decision to continue the MITR’s success as a nuclear research facility. In 1969 Thompson accepted a two-year term appointment as a U.S. atomic energy commissioner and requested Lanning to return to MIT to take his place during his temporary absence. Thompson initiated feasibility studies for a new MITR core design and believed Lanning was the most capable person to continue the task of seeing the MITR redesign to fruition.
Lanning returned to MIT in July 1969 with a faculty appointment to take over the subjects Thompson was teaching, in addition to being co-director of the MITR with Lincoln Clark Jr. during the redesign. Tragically, Thompson was killed in a plane accident in November 1970, just one week after Lanning and his team submitted the application for the redesign’s construction permit.
Thompson’s death meant his responsibilities were now Lanning’s on a permanent basis. Lanning continued to completion the redesign of the MITR, known today as the MITR-II. The redesign increased the neutron flux level by a factor of three without changing its operating power — expanding the reactor’s research capabilities and refreshing its status as a premier research facility.
Construction and startup tests for the MITR-II were completed in 1975 and the MITR-II went critical on Aug. 14, 1975. Management of the MITR-II was transferred the following year from the Nuclear Engineering Department to its own interdepartmental research center, the Nuclear Reactor Laboratory , where Lanning continued to use the MITR-II for research.
Beyond the redesign
In 1970, Lanning combined two reactor design courses he inherited and introduced a new course in which he had students apply their knowledge and critique the design and economic considerations of a reactor presented by a student in a prior term. He taught these courses through the late 1990s, in addition to leading new courses with other faculty for industry professionals on reactor safety.
Co-author of over 70 papers , many on the forefront of nuclear engineering, Lanning’s research included studies to improve the efficiency, cycle management, and design of nuclear fuel, as well as making reactors safer and more economical to operate.
Lanning was part of an ongoing research project team that introduced and demonstrated digital control and automation in nuclear reactor control mechanisms before any of the sort were found in reactors in the United States. Their research improved the regulatory barriers preventing commercial plants from replacing aging analog reactor control components with digital ones. The project also demonstrated that reactor operations would be more reliable, safe, and economical by introducing automation in certain reactor control systems. This led to the MITR being one of the first reactors in the United States licensed to operate using digital technology to control reactor power.
Lanning became professor emeritus in May 1989 and retired in 1994, but continued his passion for teaching through the late 1990s as a thesis advisor and reader. His legacy lives on in the still-operational MITR-II, with his former students following in his footsteps by working on fuel studies for the next version of the MITR core.
Lanning is predeceased by his wife of 60 years, Gloria Lanning, and is survived by his two children, a brother, and his many grandchildren .
Share this news article on:
Related links.
- MIT Nuclear Reactor Laboratory
- Department of Nuclear Science and Engineering
Related Topics
- Nuclear science and engineering
- Nuclear power and reactors
Related Articles
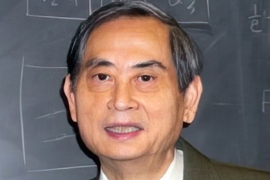
Professor Emeritus Sow-Hsin Chen, global expert in neutron science and devoted mentor, dies at 86
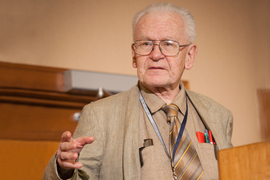
Professor Emeritus Michael Driscoll, leader in nuclear engineering and beloved mentor, dies at 86
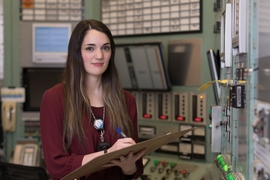
Sara Hauptman: Learning to operate a nuclear reactor
Retirement dinner honors 155 community members.
Previous item Next item
More MIT News
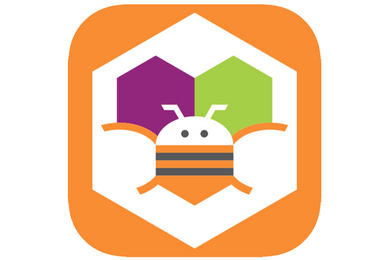
The power of App Inventor: Democratizing possibilities for mobile applications
Read full story →
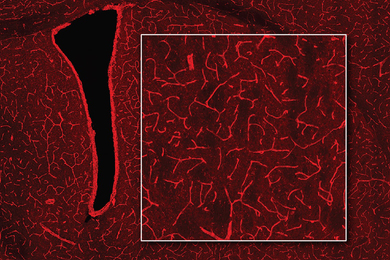
Using MRI, engineers have found a way to detect light deep in the brain
From steel engineering to ovarian tumor research
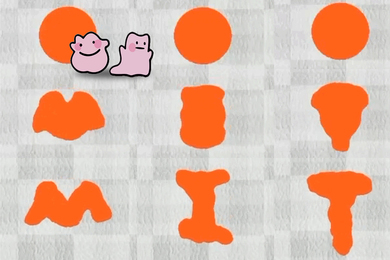
A better way to control shape-shifting soft robots
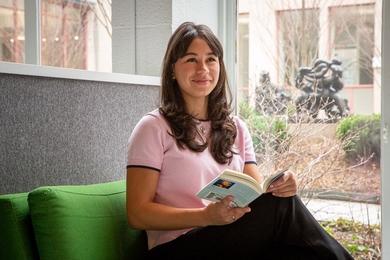
Discovering community and cultural connections
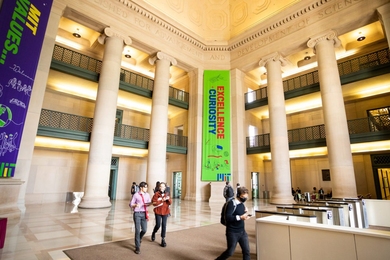
MIT Supply Chain Management Program earns top honors in three 2024 rankings
- More news on MIT News homepage →
Massachusetts Institute of Technology 77 Massachusetts Avenue, Cambridge, MA, USA
- Map (opens in new window)
- Events (opens in new window)
- People (opens in new window)
- Careers (opens in new window)
- Accessibility
- Social Media Hub
- MIT on Facebook
- MIT on YouTube
- MIT on Instagram
Find Info For
- Current Students
- Prospective Students
- Research and Partnerships
- Entrepreneurship and Commercialization
Quick Links
- Health and Life Sciences
- Info Security and AI
- Transformative Education
- Purdue Today
- Purdue Global
- Purdue in the News
May 2, 2024
Purdue University chosen by Indiana Office of Energy Development for small modular nuclear reactor study
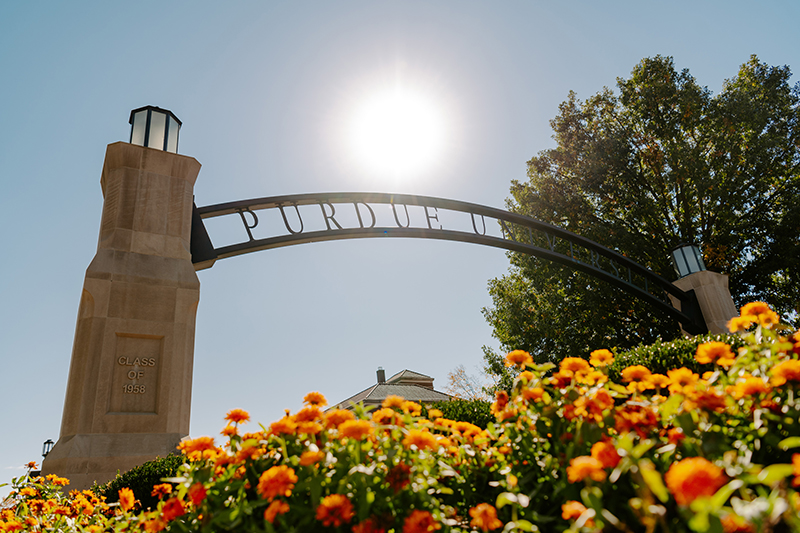
A Purdue University-led team was selected by the Indiana Office of Energy Development as the successful respondent to a request for proposals for a study to research small modular nuclear reactor technology and the potential impacts should the technology be deployed in Indiana. (Purdue University photo/Kelsey Lefever)
WEST LAFAYETTE, Ind. — Purdue University has been selected by the Indiana Office of Energy Development (IOED) to research small modular nuclear reactor (SMR) technology and analyze the potential impacts should the technology be deployed in Indiana. This partnership comes following Purdue’s selection as the successful respondent to IOED’s Request for Proposals for an Indiana-focused SMR study.
“The energy transition is ongoing and will be for decades to come. In Indiana, we’ve added a lot of tools to our toolbox to help better manage the energy transition, but the conversations must continue,” said Ryan Hadley, executive director, Indiana Office of Energy Development. “This SMR study is reflective of a proactive spirit to learn more about Indiana’s possible energy future. We are eager to learn more from this opportunity.”
The SMR study aligns with the IOED’s mission to provide comprehensive energy planning and policy development for Indiana that is affordable, stable, reliable and inclusive of a diverse and balanced generation mix.
“As a state and national leader in nuclear engineering education and research, along with our proven track record in innovation and energy generation, we are uniquely positioned to work with the Office of Energy Development on this exciting endeavor,” said Dr. Seungjin Kim, the Capt. James F. McCarthy, Jr. and Cheryl E. McCarthy Head of the School of Nuclear Engineering at Purdue University, who is leading the study as the principal investigator (PI). “We carefully assembled a project team that consists of experts from Purdue University as well as Argonne National Laboratory, Energy Systems Network and Ivy Tech Community College.”
The assembled team, in coordination with the IOED, will perform an extensive study on topics related to SMR technology:
- Current status of SMR technology
- State and local economic impact
- Workforce development and employment
- Safety, environmental impact and nuclear waste, siting considerations
- Community engagement needs and best practices
Interest in SMR technology in Indiana has grown in recent years. The Indiana General Assembly has passed legislation related to SMR technology development ( IC 8-1-8.5-12.1 ), and Purdue and Duke Energy published an interim report related to their ongoing study investigating the feasibility of using advanced nuclear energy to meet the West Lafayette campus community’s long-term energy needs. Through collaboration with some of the leading experts in advanced nuclear technology in both the public and private sector, Purdue and Duke Energy identified a number of findings and recommended next steps in the interim report, which are succinctly summarized in a two-page fact sheet .
“Purdue began seriously researching the practical application of small modular nuclear technology with Duke Energy in 2022 when we kicked off our joint feasibility study,” said Ryan Gallagher, associate vice president of Purdue Facilities Operations and Environmental Health and Safety and a co-PI for the project. “Since then, we have continued to expand our knowledge on topics including technology development, approval and implementation timelines, siting considerations and other factors that position us well to help the state evaluate the potential benefits SMRs could bring to Indiana.”
Work on the SMR study began earlier this year and will be completed by Oct. 31, 2024.
About Purdue University
Purdue University is a public research institution demonstrating excellence at scale. Ranked among top 10 public universities and with two colleges in the top four in the United States, Purdue discovers and disseminates knowledge with a quality and at a scale second to none. More than 105,000 students study at Purdue across modalities and locations, including nearly 50,000 in person on the West Lafayette campus. Committed to affordability and accessibility, Purdue’s main campus has frozen tuition 13 years in a row. See how Purdue never stops in the persistent pursuit of the next giant leap — including its first comprehensive urban campus in Indianapolis, the new Mitchell E. Daniels, Jr. School of Business, and Purdue Computes — at https://www.purdue.edu/president/strategic-initiatives .
Media Contact: Tim Doty, [email protected] .
Research News
Communication.
- OneCampus Portal
- Brightspace
- BoilerConnect
- Faculty and Staff
- Human Resources
- Colleges and Schools
Info for Staff
- Purdue Moves
- Board of Trustees
- University Senate
- Center for Healthy Living
- Information Technology
- Ethics & Compliance
- Campus Disruptions
Purdue University, 610 Purdue Mall, West Lafayette, IN 47907, (765) 494-4600
© 2015-24 Purdue University | An equal access/equal opportunity university | Copyright Complaints | Maintained by Office of Strategic Communications
Trouble with this page? Disability-related accessibility issue? Please contact News Service at [email protected] .
Cookies on GOV.UK
We use some essential cookies to make this website work.
We’d like to set additional cookies to understand how you use GOV.UK, remember your settings and improve government services.
We also use cookies set by other sites to help us deliver content from their services.
You have accepted additional cookies. You can change your cookie settings at any time.
You have rejected additional cookies. You can change your cookie settings at any time.

- Environment
- Energy infrastructure
- Low carbon technologies
UK first in Europe to invest in next generation of nuclear fuel
£196 million for high-tech nuclear fuel facility and new measures for fusion energy.
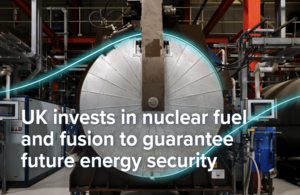
- UK to build first high-tech nuclear fuel facility in Europe to shut Putin’s Russia out of the global market and create hundreds of jobs to improve energy security at home and abroad
- high-assay low enriched uranium ( HALEU ) will power the UK’s future civil nuclear power stations, support 400 highly-skilled jobs and boost Cheshire
- announced competition for up to £600 million in contracts to build the world’s first commercially viable fusion power station prototype
The UK will be the first European nation to produce advanced nuclear fuel – a market currently dominated by Russia – to help fuel nuclear power plants at home and abroad. This is part of the government plan to push Putin out of the global energy market and drive down energy bills.
The UK will build Europe’s first facility to power future nuclear reactors - helping to isolate Russia from global energy markets, boost British energy security and provide reliable, affordable energy.
The government is awarding £196 million to Urenco to build a uranium enrichment facility. This will produce fuel by 2031 that would be ready to export or use domestically, and could power UK homes in the next decade. It will put an end to Russia’s reign as the only commercial producer of high-assay low enriched uranium ( HALEU ) and ensure other countries are not reliant on Russian exports.
The new facility will support around 400 highly-skilled jobs, helping to boost the local supply chain and grow the economy. Located at Capenhurst in Cheshire, this will cement the status of the North-West of England as a world leader in nuclear fuel production. This builds on the Prime Minister’s ‘national endeavour’ to secure the future of the UK’s thriving nuclear industry – investing at least £763 million in skills, jobs, and education.
In the 2 years since Putin illegally invaded Ukraine, the UK has led the way in cracking down on Russian oil and gas imports. Now, the UK is working with its nuclear allies to build a secure global uranium supply chain free from Russian influence.
The UK is also leading the way in fusion energy development, as engineering and construction companies will be invited on to bid for up to £600 million to build the first commercially viable fusion prototype power plant at a former coal plant in Nottinghamshire. Fusion could generate a near limitless source of clean electricity, securing the UK’s long-term energy independence.
Prime Minister, Rishi Sunak, said:
Building our own uranium enrichment plant is essential if we want to prise Putin’s blood-soaked hands off Europe’s energy market. Russia has been the sole provider of this powerful nuclear fuel for too long and this marks the latest step in pushing him out of the energy market entirely. The wider future of British nuclear remains a critical national endeavour – guaranteeing nuclear and energy security, and reducing energy bills for Brits.
Secretary of State for Energy Security and Net Zero, Claire Coutinho, said:
We stood up to Putin on oil and gas, and we won’t let him hold us to ransom on nuclear fuel. Backing Urenco to build a uranium enrichment plant here in the UK will mean we are the first European nation outside Russia to produce advanced nuclear fuel. This will support hundreds of new jobs, bring investment for the people in Cheshire and is a huge win for energy security at home and abroad.
HALEU is needed to power most advanced modular reactors which are crucial to meeting the UK’s ambition to quadruple nuclear capacity by 2050 – the biggest expansion in 70 years. Like small modular reactors, they can be made in factories and transform how power stations are built by making construction faster and less expensive.
These advanced reactors are more efficient and use novel fuels, coolants, and technologies to generate low-carbon electricity. Their high heat output means they can also be used to decarbonise industry, produce hydrogen for transport or heat for homes.
Meanwhile, the UK was the first country in the world to legislate for commercial fusion regulation, giving companies the confidence to invest here in the UK. New simpler planning measures will provide certainty to industry and strengthen the UK as a competitive location for companies to invest – putting the nation on the front foot before the technology is ready to be deployed.
A consultation launched today proposes designating all fusion plants nationally significant infrastructure projects that will be assessed by the Planning Inspectorate and ultimately decided on by the Secretary of State for energy.
Fusion power creates nearly 4 million times more energy for every kilogram of fuel than burning coal, oil or gas. Investment in the fusion technology of the future will help to create jobs, grow the economy, and strengthen the country’s energy security – delivering a cleaner energy system that will benefit future generations and bring the UK even closer to connecting fusion energy to the grid by the 2040s.
Separately, investment in high-tech nuclear fuel will unlock the market for advanced nuclear reactors and help the UK’s allies to build up their own nuclear capabilities without relying on Russia – bolstering Western energy security.
Urenco’s facility will have the capacity to produce up to 10 tonnes of HALEU per year by 2031. When fabricated into fuel, 10 tonnes of HALEU could contain as much energy as over one million tons of coal.
The funding is part of the £300 million HALEU programme announced in January this year. Urenco, which is part owned by the UK government and renowned for nuclear enrichment services, will co-fund the facility.
The programme builds on commitments made at COP28 which saw the G7 nuclear nations or ‘Sapporo 5’ - Canada, Japan, France, the UK and US – commit to increasing uranium production, as they are responsible for 50% of the world’s nuclear fuel conversion and enrichment capability.
Boris Schucht, CEO of Urenco, said:
The responsibility the nuclear industry has to help governments and customers to achieve climate change and energy security goals is clear. We welcome this government investment, which will help accelerate the development of a civil HALEU commercial market and in turn the development of the next generation of nuclear power plants. These plants will have even higher safety standards and lend themselves to quicker licensing and construction processes. Urenco has the knowledge and experience to play a leading role in the production of HALEU and other advanced fuels, operating securely under inter-governmental treaties to ensure the peaceful use and safeguarding of nuclear technology.
Paul Methven, CEO , UK Industrial Fusion Solutions, responsible for the delivery of the Spherical Tokamak for Energy Production ( STEP ), said:
We are looking towards a very significant milestone for STEP in the next 2 weeks as we are set to launch our search for industrial partners in engineering and construction who will join us in designing and delivering the STEP prototype plant at West Burton. This will demonstrate that fusion energy can work, and through that endeavour, we will develop an industry that can deliver commercial fusion for decades beyond. The launch of formal consultation on a National Policy Statement for fusion energy is very welcome and an important milestone in the journey towards a new energy source, deployed in the right way. It reflects the UK’s proactive leadership in fusion energy by putting in place the critical enablers that all fusion developments will need, and in a way that will bring communities and the public along.
Zara Hodgson, Director of the Dalton Institute, said:
This is the biggest single investment in UK nuclear fuel production capability in decades, and it is especially welcome as it will accelerate the supply of the next generation of fuels that are vital for this new net zero nuclear era. Urenco Capenhurst’s HALEU Enrichment capability will help hugely to unlock the deliverability of advanced nuclear projects, opening the door to sustainable electricity and heat for industries from nuclear, across the UK and overseas. North West of England is the home of the UK’s fuel production capability, and the Dalton Nuclear Institute celebrates this HMG and Urenco partnership that will be a catalyst for nuclear skills here and across the UK supply chain. We look now towards to how we can support this important project through training and innovation.
Tom Greatrex, Chief Executive of the Nuclear Industry Association said:
This investment will enable the UK to fuel advanced reactors around the world, building on our existing capabilities to strengthen energy security for our allies while reducing their reliance on Russia. Urenco at Capenhurst is at the very forefront of the UK’s capability, with this new facility bringing opportunities for the supply chain, new jobs and investment in the North West of England.
Notes to editors
The remaining funding will be allocated later this year to support deconversion capability, which after enrichment converts it into a form to be made into fuel, research into these innovative nuclear fuels, and capability for regulation and transport.
The procurement for STEP will be launched on 22 May.
The funding for HALEU is subject to final approvals.
Consultation: Fusion energy facilities: new National Policy Statement and proposals on siting
Share this page
The following links open in a new tab
- Share on Facebook (opens in new tab)
- Share on Twitter (opens in new tab)
Is this page useful?
- Yes this page is useful
- No this page is not useful
Help us improve GOV.UK
Don’t include personal or financial information like your National Insurance number or credit card details.
To help us improve GOV.UK, we’d like to know more about your visit today. Please fill in this survey .

COMMENTS
International Journal of Advanced Nuclear Reactor Design and Technology is a peer-reviewed, open access journal dedicated to creating a platform for sharing and exchanging the latest research results for the peaceful use of nuclear energy. The Editors welcome high-quality original research articles, technical notes, and review articles involving all aspects of nuclear science and technology.
In particular, integral pressurized water reactors (iPWRs) exhibit fuel costs 15% to 70% higher than large light water reactors, as per the 2014 nuclear fuels market data [15]. Thermodynamic ...
This paper summarizes recent research on the methods for analyzing global market potential for smaller nuclear technologies and the adaptation of these approaches to microreactors. This paper builds on a 2021 study, "Global Market Analysis of Microreactors," conducted under the DOE Microreactor Program. ... For new smaller nuclear reactors ...
Research reactor power ratings are designated in megawatts and their output can range from zero (e.g., critical assembly) up to 200 MW(th), compared with 3000 MW(th) (i.e., 1000 MW(e)) for a typical large power reactor unit. Research reactors For more than 60 years, research reactors have been providing a source of neutrons for a
The reactor core used in this AI design optimization is based on a simplification of the design of the actual TCR core. The core is a right cylinder that is 1 m in diameter and 80 cm tall. Nine ...
With the innovation of nuclear energy technology, Generation IV reactors, small nuclear reactors, and fusion reactors have received widespread attention and research. The Generation IV reactors, such as ultra-high temperature reactors, liquid metal fast reactors, molten salt reactors, etc., have made significant improvements in sustainability, safety, economy, and suppression of nuclear ...
Much current research focuses on development of safe, competitive, and sustainable nuclear energy technologies in a post Fukushima world. This special issue of Energies focuses on advances with the potential to transform contemporary reactor technologies or advanced nuclear energy systems and fuel cycles. Technologies considered in this special ...
Nuclear energy is presented as a real option in the face of the current problem of climate change and the need to reduce CO2 emissions. The nuclear reactor design with the greatest global impact throughout history and which has the most ambitious development plans is the Pressurized Water Reactor (PWR). Thus, a global review of such a reactor design is presented in this paper, utilizing the ...
reactors, but its capacity has been nearly flat for the past three decades.1 High capital costs, low electricity demand growth, and competition from cheaper sources of electricity, such as natural gas and renewables, have dampened the demand for new nuclear power plants and led to the permanent shutdown of existing reactors. Thirteen nuclear ...
The purpose of this paper is to present a model for nuclear safety management in an operational organization of a nuclear power plant, based on the HTOE (human, technology, organization, and ...
This publication presents an overview of research reactor capabilities and capacities in the development of fuels and materials for innovative nuclear reactors, such as GenIV reactors. The compendium provides comprehensive information on the potential for materials and fuel testing research of 30 research reactors, both operational and in ...
He earned his PhD in engineering and public policy at Carnegie Mellon University (CMU), where he conducted research in energy and the environment, with a focus on advanced reactor technology development and proliferation risk. Dr. Ford also served a full career as an officer in the United States Navy and held Navy subspecialties in nuclear ...
The researches on nuclear reactor thermal-hydraulics have achieved outstanding progresses in the past decades. In recent years, basic research on multiphase flow dynamics and corresponding measurement technology, as well as preliminary research on Gen IV reactors based on experiments and simulations are attracting more and more attention. However, the inside complicated physics and outside ...
The Fukushima crisis revealed the dangers of building multiple nuclear reactor units close to one another. This proximity triggered the parallel, chain-reaction accidents that led to hydrogen explosions blowing the roofs off of reactor buildings and water draining from open-air spent fuel pools—a situation that was potentially more dangerous ...
Research on the fourth generation reactors is needed for the realisation of this development. For the fast nuclear reactors, a substantial research and development effort is required in many fields—from material sciences to safety demonstration—to attain the envisaged goals. Fusion provides a long-term vision for an efficient energy production.
Research reactors are nuclear reactors used for research, development, education and training. They produce neutrons for use in industry, medicine, agriculture and forensics, among others. The IAEA assists Member States with the construction, operation, utilization and fuel cycle of research reactors, as well as with capacity-building and infrastructure development.
Nuclear safety research started with the Fermi reactor, where multiple redundant safety systems played important roles in keeping the whole operation under control. However, in the unexpected second nuclear era, the concept of nuclear safety has gone far beyond technology, as nuclear safety has been recognized as a prevalent social issue more ...
See also paper on Australian Research Reactors. Fuel. Research reactor fuel is more highly enriched (typically about 20% today) than power reactor fuel. It is high-assay low-enriched uranium (HALEU), which means it has less U-238, hence the used fuel has fewer actinides and less heat from radioactive decay.
He taught these courses through the late 1990s, in addition to leading new courses with other faculty for industry professionals on reactor safety. Co-author of over 70 papers, many on the forefront of nuclear engineering, Lanning's research included studies to improve the efficiency, cycle management, and design of nuclear fuel, as well as ...
Purdue University has been selected by the Indiana Office of Energy Development (IOED) to research small modular nuclear reactor (SMR) technology and analyze the potential impacts should the technology be deployed in Indiana. This partnership comes following Purdue's selection as the successful respondent to IOED's Request for Proposals for an Indiana-focused SMR study.
An "economically attractive" way to produce nuclear fusion energy using tokamak reactors could be a step closer, with a new operating method developed by scientists from China and the United ...
As the Coalition finalises a policy for coal-fired power station sites to host nuclear energy - and for small modular reactor technology to be deployed in other places - focus group research ...
HALEU is needed to power most advanced modular reactors which are crucial to meeting the UK's ambition to quadruple nuclear capacity by 2050 - the biggest expansion in 70 years. Like small ...