
How it works
Transform your enterprise with the scalable mindsets, skills, & behavior change that drive performance.
Explore how BetterUp connects to your core business systems.
We pair AI with the latest in human-centered coaching to drive powerful, lasting learning and behavior change.
Build leaders that accelerate team performance and engagement.
Unlock performance potential at scale with AI-powered curated growth journeys.
Build resilience, well-being and agility to drive performance across your entire enterprise.
Transform your business, starting with your sales leaders.
Unlock business impact from the top with executive coaching.
Foster a culture of inclusion and belonging.
Accelerate the performance and potential of your agencies and employees.
See how innovative organizations use BetterUp to build a thriving workforce.
Discover how BetterUp measurably impacts key business outcomes for organizations like yours.
A demo is the first step to transforming your business. Meet with us to develop a plan for attaining your goals.

- What is coaching?
Learn how 1:1 coaching works, who its for, and if it's right for you.
Accelerate your personal and professional growth with the expert guidance of a BetterUp Coach.
Types of Coaching
Navigate career transitions, accelerate your professional growth, and achieve your career goals with expert coaching.
Enhance your communication skills for better personal and professional relationships, with tailored coaching that focuses on your needs.
Find balance, resilience, and well-being in all areas of your life with holistic coaching designed to empower you.
Discover your perfect match : Take our 5-minute assessment and let us pair you with one of our top Coaches tailored just for you.

Research, expert insights, and resources to develop courageous leaders within your organization.
Best practices, research, and tools to fuel individual and business growth.
View on-demand BetterUp events and learn about upcoming live discussions.
The latest insights and ideas for building a high-performing workplace.
- BetterUp Briefing
The online magazine that helps you understand tomorrow's workforce trends, today.
Innovative research featured in peer-reviewed journals, press, and more.
Founded in 2022 to deepen the understanding of the intersection of well-being, purpose, and performance
We're on a mission to help everyone live with clarity, purpose, and passion.
Join us and create impactful change.
Read the buzz about BetterUp.
Meet the leadership that's passionate about empowering your workforce.

For Business
For Individuals

How to write a speech that your audience remembers
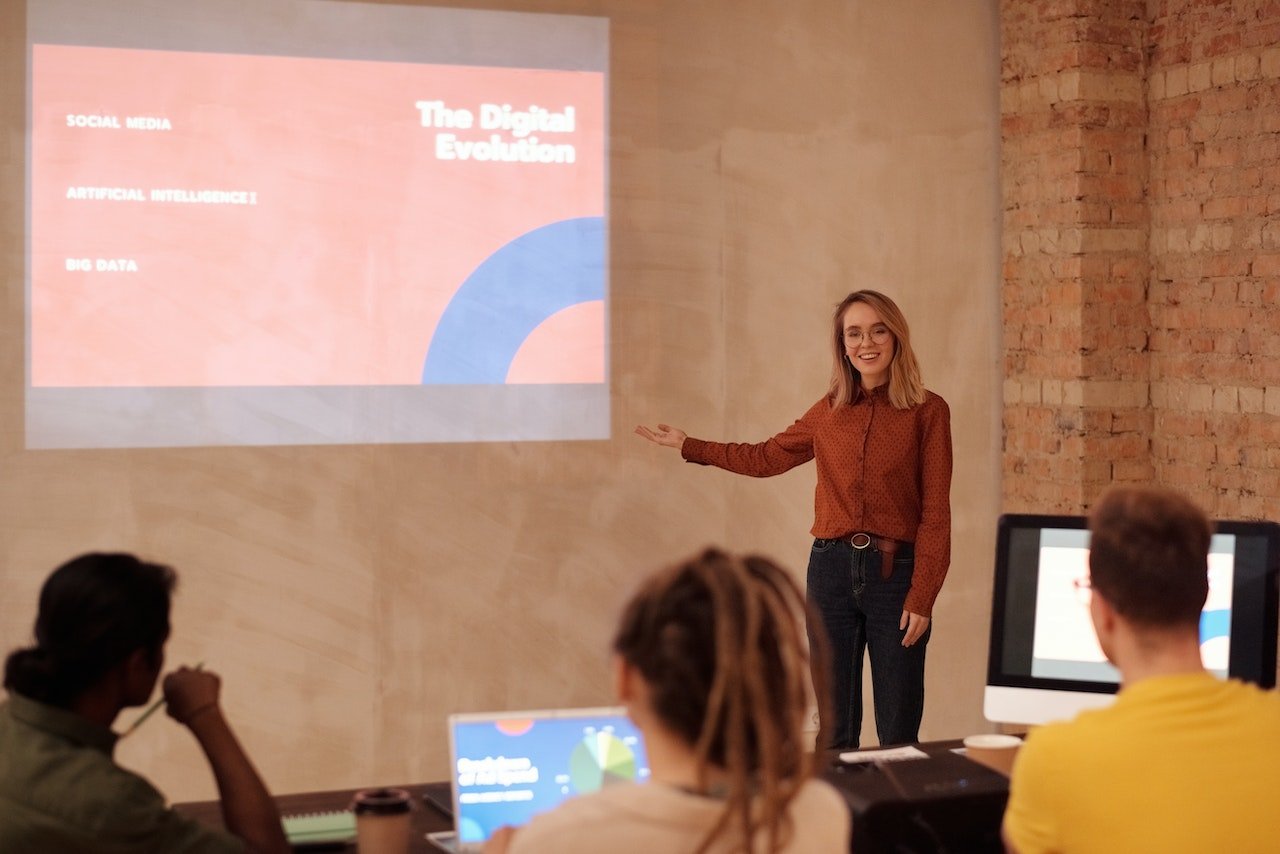
Elevate your communication skills
Unlock the power of clear and persuasive communication. Our coaches can guide you to build strong relationships and succeed in both personal and professional life.
Whether in a work meeting or at an investor panel, you might give a speech at some point. And no matter how excited you are about the opportunity, the experience can be nerve-wracking .
But feeling butterflies doesn’t mean you can’t give a great speech. With the proper preparation and a clear outline, apprehensive public speakers and natural wordsmiths alike can write and present a compelling message. Here’s how to write a good speech you’ll be proud to deliver.
What is good speech writing?
Good speech writing is the art of crafting words and ideas into a compelling, coherent, and memorable message that resonates with the audience. Here are some key elements of great speech writing:
- It begins with clearly understanding the speech's purpose and the audience it seeks to engage.
- A well-written speech clearly conveys its central message, ensuring that the audience understands and retains the key points.
- It is structured thoughtfully, with a captivating opening, a well-organized body, and a conclusion that reinforces the main message.
- Good speech writing embraces the power of engaging content, weaving in stories, examples, and relatable anecdotes to connect with the audience on both intellectual and emotional levels.
Ultimately, it is the combination of these elements, along with the authenticity and delivery of the speaker , that transforms words on a page into a powerful and impactful spoken narrative.
What makes a good speech?
A great speech includes several key qualities, but three fundamental elements make a speech truly effective:
Clarity and purpose
Remembering the audience, cohesive structure.
While other important factors make a speech a home run, these three elements are essential for writing an effective speech.
The main elements of a good speech
The main elements of a speech typically include:
- Introduction: The introduction sets the stage for your speech and grabs the audience's attention. It should include a hook or attention-grabbing opening, introduce the topic, and provide an overview of what will be covered.
- Opening/captivating statement: This is a strong statement that immediately engages the audience and creates curiosity about the speech topics.
- Thesis statement/central idea: The thesis statement or central idea is a concise statement that summarizes the main point or argument of your speech. It serves as a roadmap for the audience to understand what your speech is about.
- Body: The body of the speech is where you elaborate on your main points or arguments. Each point is typically supported by evidence, examples, statistics, or anecdotes. The body should be organized logically and coherently, with smooth transitions between the main points.
- Supporting evidence: This includes facts, data, research findings, expert opinions, or personal stories that support and strengthen your main points. Well-chosen and credible evidence enhances the persuasive power of your speech.
- Transitions: Transitions are phrases or statements that connect different parts of your speech, guiding the audience from one idea to the next. Effective transitions signal the shifts in topics or ideas and help maintain a smooth flow throughout the speech.
- Counterarguments and rebuttals (if applicable): If your speech involves addressing opposing viewpoints or counterarguments, you should acknowledge and address them. Presenting counterarguments makes your speech more persuasive and demonstrates critical thinking.
- Conclusion: The conclusion is the final part of your speech and should bring your message to a satisfying close. Summarize your main points, restate your thesis statement, and leave the audience with a memorable closing thought or call to action.
- Closing statement: This is the final statement that leaves a lasting impression and reinforces the main message of your speech. It can be a call to action, a thought-provoking question, a powerful quote, or a memorable anecdote.
- Delivery and presentation: How you deliver your speech is also an essential element to consider. Pay attention to your tone, body language, eye contact , voice modulation, and timing. Practice and rehearse your speech, and try using the 7-38-55 rule to ensure confident and effective delivery.
While the order and emphasis of these elements may vary depending on the type of speech and audience, these elements provide a framework for organizing and delivering a successful speech.

How to structure a good speech
You know what message you want to transmit, who you’re delivering it to, and even how you want to say it. But you need to know how to start, develop, and close a speech before writing it.
Think of a speech like an essay. It should have an introduction, conclusion, and body sections in between. This places ideas in a logical order that the audience can better understand and follow them. Learning how to make a speech with an outline gives your storytelling the scaffolding it needs to get its point across.
Here’s a general speech structure to guide your writing process:
- Explanation 1
- Explanation 2
- Explanation 3
How to write a compelling speech opener
Some research shows that engaged audiences pay attention for only 15 to 20 minutes at a time. Other estimates are even lower, citing that people stop listening intently in fewer than 10 minutes . If you make a good first impression at the beginning of your speech, you have a better chance of interesting your audience through the middle when attention spans fade.
Implementing the INTRO model can help grab and keep your audience’s attention as soon as you start speaking. This acronym stands for interest, need, timing, roadmap, and objectives, and it represents the key points you should hit in an opening.
Here’s what to include for each of these points:
- Interest : Introduce yourself or your topic concisely and speak with confidence . Write a compelling opening statement using relevant data or an anecdote that the audience can relate to.
- Needs : The audience is listening to you because they have something to learn. If you’re pitching a new app idea to a panel of investors, those potential partners want to discover more about your product and what they can earn from it. Read the room and gently remind them of the purpose of your speech.
- Timing : When appropriate, let your audience know how long you’ll speak. This lets listeners set expectations and keep tabs on their own attention span. If a weary audience member knows you’ll talk for 40 minutes, they can better manage their energy as that time goes on.
- Routemap : Give a brief overview of the three main points you’ll cover in your speech. If an audience member’s attention starts to drop off and they miss a few sentences, they can more easily get their bearings if they know the general outline of the presentation.
- Objectives : Tell the audience what you hope to achieve, encouraging them to listen to the end for the payout.
Writing the middle of a speech
The body of your speech is the most information-dense section. Facts, visual aids, PowerPoints — all this information meets an audience with a waning attention span. Sticking to the speech structure gives your message focus and keeps you from going off track, making everything you say as useful as possible.
Limit the middle of your speech to three points, and support them with no more than three explanations. Following this model organizes your thoughts and prevents you from offering more information than the audience can retain.
Using this section of the speech to make your presentation interactive can add interest and engage your audience. Try including a video or demonstration to break the monotony. A quick poll or survey also keeps the audience on their toes.
Wrapping the speech up
To you, restating your points at the end can feel repetitive and dull. You’ve practiced countless times and heard it all before. But repetition aids memory and learning , helping your audience retain what you’ve told them. Use your speech’s conclusion to summarize the main points with a few short sentences.
Try to end on a memorable note, like posing a motivational quote or a thoughtful question the audience can contemplate once they leave. In proposal or pitch-style speeches, consider landing on a call to action (CTA) that invites your audience to take the next step.

How to write a good speech
If public speaking gives you the jitters, you’re not alone. Roughly 80% of the population feels nervous before giving a speech, and another 10% percent experiences intense anxiety and sometimes even panic.
The fear of failure can cause procrastination and can cause you to put off your speechwriting process until the last minute. Finding the right words takes time and preparation, and if you’re already feeling nervous, starting from a blank page might seem even harder.
But putting in the effort despite your stress is worth it. Presenting a speech you worked hard on fosters authenticity and connects you to the subject matter, which can help your audience understand your points better. Human connection is all about honesty and vulnerability, and if you want to connect to the people you’re speaking to, they should see that in you.
1. Identify your objectives and target audience
Before diving into the writing process, find healthy coping strategies to help you stop worrying . Then you can define your speech’s purpose, think about your target audience, and start identifying your objectives. Here are some questions to ask yourself and ground your thinking :
- What purpose do I want my speech to achieve?
- What would it mean to me if I achieved the speech’s purpose?
- What audience am I writing for?
- What do I know about my audience?
- What values do I want to transmit?
- If the audience remembers one take-home message, what should it be?
- What do I want my audience to feel, think, or do after I finish speaking?
- What parts of my message could be confusing and require further explanation?
2. Know your audience
Understanding your audience is crucial for tailoring your speech effectively. Consider the demographics of your audience, their interests, and their expectations. For instance, if you're addressing a group of healthcare professionals, you'll want to use medical terminology and data that resonate with them. Conversely, if your audience is a group of young students, you'd adjust your content to be more relatable to their experiences and interests.
3. Choose a clear message
Your message should be the central idea that you want your audience to take away from your speech. Let's say you're giving a speech on climate change. Your clear message might be something like, "Individual actions can make a significant impact on mitigating climate change." Throughout your speech, all your points and examples should support this central message, reinforcing it for your audience.
4. Structure your speech
Organizing your speech properly keeps your audience engaged and helps them follow your ideas. The introduction should grab your audience's attention and introduce the topic. For example, if you're discussing space exploration, you could start with a fascinating fact about a recent space mission. In the body, you'd present your main points logically, such as the history of space exploration, its scientific significance, and future prospects. Finally, in the conclusion, you'd summarize your key points and reiterate the importance of space exploration in advancing human knowledge.
5. Use engaging content for clarity
Engaging content includes stories, anecdotes, statistics, and examples that illustrate your main points. For instance, if you're giving a speech about the importance of reading, you might share a personal story about how a particular book changed your perspective. You could also include statistics on the benefits of reading, such as improved cognitive abilities and empathy.
6. Maintain clarity and simplicity
It's essential to communicate your ideas clearly. Avoid using overly technical jargon or complex language that might confuse your audience. For example, if you're discussing a medical breakthrough with a non-medical audience, explain complex terms in simple, understandable language.
7. Practice and rehearse
Practice is key to delivering a great speech. Rehearse multiple times to refine your delivery, timing, and tone. Consider using a mirror or recording yourself to observe your body language and gestures. For instance, if you're giving a motivational speech, practice your gestures and expressions to convey enthusiasm and confidence.
8. Consider nonverbal communication
Your body language, tone of voice, and gestures should align with your message . If you're delivering a speech on leadership, maintain strong eye contact to convey authority and connection with your audience. A steady pace and varied tone can also enhance your speech's impact.
9. Engage your audience
Engaging your audience keeps them interested and attentive. Encourage interaction by asking thought-provoking questions or sharing relatable anecdotes. If you're giving a speech on teamwork, ask the audience to recall a time when teamwork led to a successful outcome, fostering engagement and connection.
10. Prepare for Q&A
Anticipate potential questions or objections your audience might have and prepare concise, well-informed responses. If you're delivering a speech on a controversial topic, such as healthcare reform, be ready to address common concerns, like the impact on healthcare costs or access to services, during the Q&A session.
By following these steps and incorporating examples that align with your specific speech topic and purpose, you can craft and deliver a compelling and impactful speech that resonates with your audience.

Tools for writing a great speech
There are several helpful tools available for speechwriting, both technological and communication-related. Here are a few examples:
- Word processing software: Tools like Microsoft Word, Google Docs, or other word processors provide a user-friendly environment for writing and editing speeches. They offer features like spell-checking, grammar correction, formatting options, and easy revision tracking.
- Presentation software: Software such as Microsoft PowerPoint or Google Slides is useful when creating visual aids to accompany your speech. These tools allow you to create engaging slideshows with text, images, charts, and videos to enhance your presentation.
- Speechwriting Templates: Online platforms or software offer pre-designed templates specifically for speechwriting. These templates provide guidance on structuring your speech and may include prompts for different sections like introductions, main points, and conclusions.
- Rhetorical devices and figures of speech: Rhetorical tools such as metaphors, similes, alliteration, and parallelism can add impact and persuasion to your speech. Resources like books, websites, or academic papers detailing various rhetorical devices can help you incorporate them effectively.
- Speechwriting apps: Mobile apps designed specifically for speechwriting can be helpful in organizing your thoughts, creating outlines, and composing a speech. These apps often provide features like voice recording, note-taking, and virtual prompts to keep you on track.
- Grammar and style checkers: Online tools or plugins like Grammarly or Hemingway Editor help improve the clarity and readability of your speech by checking for grammar, spelling, and style errors. They provide suggestions for sentence structure, word choice, and overall tone.
- Thesaurus and dictionary: Online or offline resources such as thesauruses and dictionaries help expand your vocabulary and find alternative words or phrases to express your ideas more effectively. They can also clarify meanings or provide context for unfamiliar terms.
- Online speechwriting communities: Joining online forums or communities focused on speechwriting can be beneficial for getting feedback, sharing ideas, and learning from experienced speechwriters. It's an opportunity to connect with like-minded individuals and improve your public speaking skills through collaboration.
Remember, while these tools can assist in the speechwriting process, it's essential to use them thoughtfully and adapt them to your specific needs and style. The most important aspect of speechwriting remains the creativity, authenticity, and connection with your audience that you bring to your speech.

5 tips for writing a speech
Behind every great speech is an excellent idea and a speaker who refined it. But a successful speech is about more than the initial words on the page, and there are a few more things you can do to help it land.
Here are five more tips for writing and practicing your speech:
1. Structure first, write second
If you start the writing process before organizing your thoughts, you may have to re-order, cut, and scrap the sentences you worked hard on. Save yourself some time by using a speech structure, like the one above, to order your talking points first. This can also help you identify unclear points or moments that disrupt your flow.
2. Do your homework
Data strengthens your argument with a scientific edge. Research your topic with an eye for attention-grabbing statistics, or look for findings you can use to support each point. If you’re pitching a product or service, pull information from company metrics that demonstrate past or potential successes.
Audience members will likely have questions, so learn all talking points inside and out. If you tell investors that your product will provide 12% returns, for example, come prepared with projections that support that statement.
3. Sound like yourself
Memorable speakers have distinct voices. Think of Martin Luther King Jr’s urgent, inspiring timbre or Oprah’s empathetic, personal tone . Establish your voice — one that aligns with your personality and values — and stick with it. If you’re a motivational speaker, keep your tone upbeat to inspire your audience . If you’re the CEO of a startup, try sounding assured but approachable.
4. Practice
As you practice a speech, you become more confident , gain a better handle on the material, and learn the outline so well that unexpected questions are less likely to trip you up. Practice in front of a colleague or friend for honest feedback about what you could change, and speak in front of the mirror to tweak your nonverbal communication and body language .
5. Remember to breathe
When you’re stressed, you breathe more rapidly . It can be challenging to talk normally when you can’t regulate your breath. Before your presentation, try some mindful breathing exercises so that when the day comes, you already have strategies that will calm you down and remain present . This can also help you control your voice and avoid speaking too quickly.
How to ghostwrite a great speech for someone else
Ghostwriting a speech requires a unique set of skills, as you're essentially writing a piece that will be delivered by someone else. Here are some tips on how to effectively ghostwrite a speech:
- Understand the speaker's voice and style : Begin by thoroughly understanding the speaker's personality, speaking style, and preferences. This includes their tone, humor, and any personal anecdotes they may want to include.
- Interview the speaker : Have a detailed conversation with the speaker to gather information about their speech's purpose, target audience, key messages, and any specific points they want to emphasize. Ask for personal stories or examples they may want to include.
- Research thoroughly : Research the topic to ensure you have a strong foundation of knowledge. This helps you craft a well-informed and credible speech.
- Create an outline : Develop a clear outline that includes the introduction, main points, supporting evidence, and a conclusion. Share this outline with the speaker for their input and approval.
- Write in the speaker's voice : While crafting the speech, maintain the speaker's voice and style. Use language and phrasing that feel natural to them. If they have a particular way of expressing ideas, incorporate that into the speech.
- Craft a captivating opening : Begin the speech with a compelling opening that grabs the audience's attention. This could be a relevant quote, an interesting fact, a personal anecdote, or a thought-provoking question.
- Organize content logically : Ensure the speech flows logically, with each point building on the previous one. Use transitions to guide the audience from one idea to the next smoothly.
- Incorporate engaging stories and examples : Include anecdotes, stories, and real-life examples that illustrate key points and make the speech relatable and memorable.
- Edit and revise : Edit the speech carefully for clarity, grammar, and coherence. Ensure the speech is the right length and aligns with the speaker's time constraints.
- Seek feedback : Share drafts of the speech with the speaker for their feedback and revisions. They may have specific changes or additions they'd like to make.
- Practice delivery : If possible, work with the speaker on their delivery. Practice the speech together, allowing the speaker to become familiar with the content and your writing style.
- Maintain confidentiality : As a ghostwriter, it's essential to respect the confidentiality and anonymity of the work. Do not disclose that you wrote the speech unless you have the speaker's permission to do so.
- Be flexible : Be open to making changes and revisions as per the speaker's preferences. Your goal is to make them look good and effectively convey their message.
- Meet deadlines : Stick to agreed-upon deadlines for drafts and revisions. Punctuality and reliability are essential in ghostwriting.
- Provide support : Support the speaker during their preparation and rehearsal process. This can include helping with cue cards, speech notes, or any other materials they need.
Remember that successful ghostwriting is about capturing the essence of the speaker while delivering a well-structured and engaging speech. Collaboration, communication, and adaptability are key to achieving this.
Give your best speech yet
Learn how to make a speech that’ll hold an audience’s attention by structuring your thoughts and practicing frequently. Put the effort into writing and preparing your content, and aim to improve your breathing, eye contact , and body language as you practice. The more you work on your speech, the more confident you’ll become.
The energy you invest in writing an effective speech will help your audience remember and connect to every concept. Remember: some life-changing philosophies have come from good speeches, so give your words a chance to resonate with others. You might even change their thinking.
Elizabeth Perry, ACC
Elizabeth Perry is a Coach Community Manager at BetterUp. She uses strategic engagement strategies to cultivate a learning community across a global network of Coaches through in-person and virtual experiences, technology-enabled platforms, and strategic coaching industry partnerships. With over 3 years of coaching experience and a certification in transformative leadership and life coaching from Sofia University, Elizabeth leverages transpersonal psychology expertise to help coaches and clients gain awareness of their behavioral and thought patterns, discover their purpose and passions, and elevate their potential. She is a lifelong student of psychology, personal growth, and human potential as well as an ICF-certified ACC transpersonal life and leadership Coach.
10 examples of principles that can guide your approach to work
The 11 tips that will improve your public speaking skills, create a networking plan in 7 easy steps, 10+ interpersonal skills at work and ways to develop them, make the connection: 10 effective ways to connect with people, are you being passed over for a promotion here’s what to do, how to write an impactful cover letter for a career change, what are analytical skills examples and how to level up, what is a career statement, and should you write one, similar articles, how to write an executive summary in 10 steps, the importance of good speech: 5 tips to be more articulate, how to pitch ideas: 8 tips to captivate any audience, how to give a good presentation that captivates any audience, anxious about meetings learn how to run a meeting with these 10 tips, writing an elevator pitch about yourself: a how-to plus tips, how to make a presentation interactive and exciting, 6 presentation skills and how to improve them, how to write a memo: 8 steps with examples, stay connected with betterup, get our newsletter, event invites, plus product insights and research..
3100 E 5th Street, Suite 350 Austin, TX 78702
- Platform Overview
- Integrations
- Powered by AI
- BetterUp Lead
- BetterUp Manage™
- BetterUp Care™
- Sales Performance
- Diversity & Inclusion
- Case Studies
- Why BetterUp?
- About Coaching
- Find your Coach
- Career Coaching
- Communication Coaching
- Life Coaching
- News and Press
- Leadership Team
- Become a BetterUp Coach
- BetterUp Labs
- Center for Purpose & Performance
- Leadership Training
- Business Coaching
- Contact Support
- Contact Sales
- Privacy Policy
- Acceptable Use Policy
- Trust & Security
- Cookie Preferences

Student How To Start A Speech
The opening of a speech typically includes elements such as greeting the audience, introducing the speaker, expressing appreciation, and an attention-grabbing introduction. Here you will get a guide on Student how to start a speech.
Starting a speech may include setting the purpose of the speech, determining its relevance to the audience, outlining the structure of the presentation, and creating a positive and enthusiastic atmosphere.
How to start a speech as a student?
Starting your speech effectively is very important to grab your audience’s attention and set the mood for the rest of your presentation.
Here are some steps and tips on how to start a speech as a student , these are as… 1. Introduction and Greetings
Begin by introducing yourself and greeting the audience. “Good morning/afternoon/evening, everyone.
My name is [your name]. I am pleased to be here today to speak on [your topic].” 2. Engaging Opening
Captivate your audience from the start. Use compelling quotes, interesting facts, thought-provoking questions, or short anecdotes related to your topic.
This will pique their curiosity and make your speech more receptive. 3. State the Purpose
Clearly state the purpose of your speech. Let your audience know what you aim to accomplish or what they can expect to learn from your talk.
For example:
“Today I would like to share some interesting insights about [your topic] and why it is important to all of us.” 4. Establish Relevance
Create a connection between your topic and your audience. Explain why your topic is important to them or how it relates to their interests and concerns.
This creates a sense of relevance and helps keep your audience engaged. 5. Outline the Structure
Give a brief overview of the main points or sections of your speech. This will help your audience understand the structure of your presentation and prepare them mentally for what is to come.
“He describes three important aspects of [your topic]: [first point], [second point], [third point].” 6. Use Visuals or Props (if appropriate)
Depending on your topic, you can use visual aids and props to give your introduction more impact.
This can be strong images, graphics, thematic props, or short video clips. 7. Maintain Confidence and Enthusiasm
Present your ideas with confidence and enthusiasm. Smile and make eye contact with your audience to connect with them.
Being positive and confident can increase your credibility and attract your audience. 8. Keep it Brief
Your introduction should grab attention, but it should also be concise. Don’t go into too much detail here. Stores more detailed information about the body of the speech.
ALSO, READ | How to reply to thank you?
Getting off to a good start is important, but just as important is keeping your audience engaged for the rest of your speech . So practice your speech, be organized, speak passionately and clearly, and deliver engaging presentations. I wish you good luck!

Starting lines of speech for students (Speech Starting Lines in English in School)
Here are a few beginning strains for a speech focused in the direction of students…
1. Fellow students, educators, and esteemed guests, these days we acquire right here with a shared purpose – to have a good time knowledge, growth, and the boundless opportunities that lie beforehand.
2. Hello, my fellow students! As we stand on the edge of a brand new bankruptcy in our lives, I can`t assist but sense a combination of excitement, nervousness, and the fun of infinite opportunities.
3. Good [morning/afternoon], students! I am venerated to deal with this sort of colorful and dynamic audience. Each of you represents the future, and I am keen to embark on this adventure of getting to know together.
4. Dear classmates, it is an absolute delight to face earlier than you these days. We are extra than only a collection of students; we’re a network of dreamers, doers, and alternate-makers.
5. Greetings, younger minds! Today, I need to percentage with you a message that transcends the bounds of lecture rooms and textbooks, a message that empowers every folk to form our destinies.
6. Hey there, fellow students! I need to take a second to mirror the great adventure we have got been on so far, and additionally appearance beforehand to the notable adventures that watch for us.
7. Good [morning/afternoon], everyone! As students, we’re the architects of our futures, and with the proper mindset and determination, we are able to construct a global full of infinite opportunities.
8. To all my fellow students, I stand right here with gigantic satisfaction and gratitude. Our particular views and numerous capabilities have the strength to alternate the global, beginning proper right here, proper now.
9. Hello, formidable minds! Today, I invite you to sign up for me on a quest for knowledge, for it is thru getting to know that we liberate the doorways to a brighter and extra promising future.
10. Greetings to my fellow learners! Remember that the pursuit of training isn’t always pretty much grades; it is approximately obtaining wisdom, empathy, and the ability to make a wonderful effect globally.
Starting your speech with a clear and engaging introduction lays the foundation for a memorable and impactful speech. These starting lines of speech in English will help students in school .
How to start a speech of introduction?
Starting an introductory speech (student speech start) is an important task because it sets the stage for the introducer and leaves a positive impression on the audience.
“I am honored to be here today to introduce an outstanding person who has made a significant contribution to [region/industry/topic]. “
Briefly state your knowledge of your relationship with the person you are introducing or their accomplishments.
Use positive adjectives and descriptive phrases to spark enthusiasm in the person you are introducing.
Explain why the person you are introducing is relevant and valuable to your audience.
Your positive attitude is contagious and your audience will be more receptive to your upcoming speeches.
The focus should be on the speaker, not the introduction itself.
After introducing the person, gently transition to being the keynote speaker or inviting them on stage.
An effective introduction not only builds rapport with the person you are introducing but also creates a positive atmosphere for the rest of the event and speech.
Practice introducing yourself smoothly and appropriately expressing your admiration for the speaker.
Best introduction speech for students
A good entrance speech for a student (student speech start) should be engaging, inspiring, and relatable. Here is the best introduction speech for students you can consider it.
“Good morning, dear students, lecturers, and distinguished guests, Today is a day of endless possibilities and endless possibilities.
We gather here to embark on a journey of growth, learning, and discovery that will shape our future and leave a lasting impact on the world.
We all come from different backgrounds, have our own dreams, and have different talents. Nevertheless, we are united by our common quest for knowledge, our hunger for knowledge, and our passion to make a difference.
We are now on the threshold of a new chapter in our lives where the door of possibility is wide open. It’s time to build lifelong friendships, overcome challenges, and pave the way to success. But remember, you won’t be walking this journey alone. Together, we are a force to be reckoned with as a community of thinkers, innovators, and change-makers.
The classrooms, laboratories, and halls of this institution promote not only academic excellence, but the virtues of empathy, compassion, and resilience. It’s not just the grades you get that matter. It’s about the values we uphold and the impact we make. As the new school year begins, seize every opportunity in front of you.
Get out of your comfort zone and be bold to dream big. Be curious, ask questions, and seek knowledge beyond the textbook. And when difficulties inevitably arise, let us remember that we are not alone in this journey.
Our teachers, mentors, and fellow students are here to support and encourage us.
Today we stand on the threshold of greatness. This is a place where dreams come true, ideas become reality and each of us has the power to make a positive impact on the world. So, my friends, let us face this adventure together with determination in our hearts, with enthusiasm, and with the conviction that we are destined for great things.
May this year be a time of growth, a time of learning, and a chapter to look back on with pride. Let’s make it a memorable year as a turning point in your life. Thank you and let’s make it a journey for books!”
The purpose of this introductory speech is to inspire and motivate students to approach the school year with enthusiasm and determination.
It highlights the power of unity, growth, and community as they embark on their educational journey together.
Famous speech opening lines
There have been numerous famous speeches throughout history, each with memorable opening lines that captured the attention of their audiences.
Here are some iconic speech opening lines… 1. “Forty-seven years ago our ancestors envisioned freedom and brought forth a new nation on this continent, true to the principle that all men are born equal.” – Abraham Lincoln, Gettysburg Address 2. “I have a dream that one day this country will rise up and live out the true meaning of the credo: ‘We have taken these truths for granted and that all men are born equal.'” – Martin Luther King, Jr., “I Have a Dream” 3. “We chose to go to the moon and do other things in the last decade not because it’s easy, but because it’s hard.” – John F. Kennedy, Moon Speech 4. “My friends, my Romans, my brethren, lend me your ear. I have not come to honor Caesar, but to bury him.” – William Shakespeare, Julius Caesar 5. “I am not a Virginian, but an American.” – Patrick Henry, Give Me Liberty or Give Me Death 6. “Don’t ask what your country can do for you. Ask what your country can do for you.” – John F. Kennedy, Inaugural Address 7. “Today, in this auditorium and around the world, we gather to remember and honor the victims of the Holocaust.” – Elie Wiesel, Nobel Peace Prize Acceptance Speech 8. “We are confronted with a moral and spiritual crisis.” – Martin Luther King Jr., Beyond Vietnam: A Time to Break Silence These opening lines are just a few examples of how powerful rhetoric can captivate audiences, evoke emotions, and leave a lasting impact. The best speeches often start with strong, evocative openings that set the tone for the entire address.
How to start a speech examples (student speech start)
Here are some examples of different ways to start a speech… 1. Start with a Quote
“As Albert Einstein once said, ‘The only source of knowledge is experience.’ Today I stand before you to share with you my experience and the wisdom I have gained from it. 2. Begin with a Thought-Provoking Question
“Have you ever wondered what it takes to turn dreams into reality? Today, we’ll explore the key ingredients that can transform aspirations into achievements.” 3. Use an Anecdote or Personal Story
“Let’s go back to a pivotal moment in my life. It was a time of uncertainty, but it taught me a valuable lesson about perseverance and the strength of the human spirit.” 4. Start with a Startling Fact or Statistic
“Did you know that nearly one-third of all food produced globally goes to waste? Today, we’ll discuss the importance of sustainability and how each of us can make a difference.” 5. Open with Humor
“Good [morning/afternoon], everyone! Before we dive into the serious stuff, let me share a quick joke to lighten the mood: Why did the scarecrow win an award? Because he was outstanding in his field!” 6. Use a Bold Statement or Declaration
“The time has come for us to challenge the status quo and redefine what’s possible. Today, I urge you to break free from the chains of conformity and embrace innovation.” 7. Start with a Historical Reference
“In 1969, humans took their first steps on the moon. Today, we gather to celebrate not just that remarkable achievement but also the spirit of exploration that drives us forward.” 8. Open with a Relevant Current Event
“In recent news, the alarming rise in environmental disasters serves as a wake-up call for us all. Together, we must take action to protect our planet for future generations.” The way you start a speech (student speech start) sets the stage for the rest of your presentation. Choose an opening that aligns with your speech’s theme and engages the audience from the very beginning.
Share this:
- Click to share on Twitter (Opens in new window)
- Click to share on Facebook (Opens in new window)
- Click to share on LinkedIn (Opens in new window)
- Click to share on WhatsApp (Opens in new window)
- Click to share on Telegram (Opens in new window)
Leave a Comment Cancel reply
Save my name, email, and website in this browser for the next time I comment.
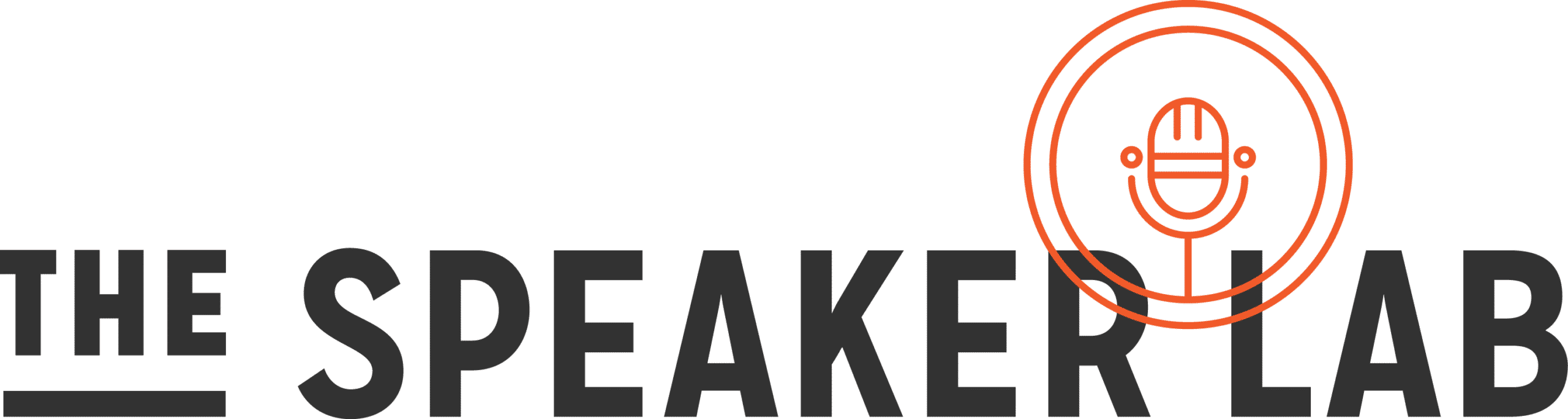
How to write a speech
- James Haynes
- October 6, 2022
Table of Contents
Introduction.
So you want to learn how to write a speech. Maybe it’s for a speech for work, or maybe it’s for a school project. You know that professional speakers don’t just make stuff up. They don’t write a few thoughts on a notecard and then shoot from the hip for an entire presentation. They take the time to write and carefully craft their material. And you’re ready to do that! You have an idea of what you want to speak about, but how do you actually create your talk? How do you give a speech? And what makes a talk “good”?
In this post, you can read answers to all of those questions. You’ll learn tips to go through the process to create a great speech from idea to completion. And you’ll learn how to write and give an inspiring speech. Ready to learn more? Read on!
Before you start to write your speech
A speech is simply a talk meant to get your audience to learn, understand or do something.
The best speakers on the planet only have one or two talks they do and those talks are insanely good. Start by developing just one, really amazing talk that resonates deeply with your intended audience. The best marketing for your speaking business is a great talk, so it is worth it to put in the hours for this part. Yes, even if your first speaking gig is a free talk at a community center.
Keep in mind: Your audience is always going to be asking two questions: “so what?” and “now what?” So what means, what does this have to do with me? Now what is what you want the audience to do as a result of your talk. Give them action steps to implement what you taught them. If they hear you speak but literally don’t do anything differently, what’s the point?
Giving a speech is almost like mapping for a road trip. If you are going to go on a road trip, it’s easier to have a paper map or Google Maps to tell you where you’re going. But if you just get in the car and you start driving, and people are in the car asking you where we’re going, you’re in trouble! But by organizing and structuring your talk, you can lead the audience to your conclusions. And you can effectively answer those two questions: “so what?” and “now what?”
Free Download: 6 Proven Steps to Book More Paid Speaking Gigs in 2024
Download our 18-page guide and start booking more paid speaking gigs today!
Want to learn how to write a speech? Read on for 3 steps to make it unforgettable:
1. Begin with the end in mind and tell a story
Have you ever been left at the end of a speech wondering, “What was the point of this talk?” Don’t do that to your audience. When creating your talk, determine the destination that you want to take them to. Once you pick a point, then you can work backwards and reverse engineer how to get your audience to that place.
The best way to do the point of your talk is to find where your audience’s needs converge with your passions. Think about what problems you like to solve and what topics you want to talk about and look out into the world. Who is asking for solutions to those problems? Become the expert on that audience and commit yourself to meeting their needs. (for more on finding your big idea, check out this episode of The Speaker Lab podcast)
Okay, so now you have your topic, the idea you want to communicate. Now what? One of the best ways to create a memorable, relatable talk is by integrating first-person stories . You don’t have to have lost a limb or scaled Mount Everest. Keep an eye out in your everyday life for little moments that can contribute to your message. Write them down and integrate them into your talk. As you get more speaking gigs, you will very quickly learn which stories are a hit and which are total flops…which is all part of the process!
Humans relate to stories. We connect to stories. Funny stories. Sad stories. Inspirational stories. We love stories. So tell them. Lots of them. Stories will keep your audience engaged and are also easier for you to memorize. Telling stories that you lived and experienced generally makes the story better for you and the audience. For the audience, they can often times find themselves in your story. For you as the speaker, it’s much easier (and more powerful) to tell a story that you lived versus one you read in a book.
2. Write out your speech from beginning to end
As Grant Baldwin discusses in this video on preparing your talk , you want to write out your talk to have a basic structure: beginning, middle, and end.
In the beginning, you’re going to want to introduce the problem that your talk is going to solve and ultimately start to capture the audience’s attention. One thing that’s important to remember is there’s a difference between an audience that wants to be there and an audience that has to be there. When you get on stage, you want to be able to answer for the audience: Who are you? Why should I pay attention? Why does this matter? What am I supposed to do with this information? Can I trust you? You want to give the audience a reason to engage with you and where you’re going with the speech.
The next part of the process is the main body. This is where you will provide the solution to the problem or elaborate on the idea you’ve presented, and then share the action items that transform the audience. These action items should be specific, tangible, actionable, and realistic. You want to give something that the audience can leave with knowing exactly what to do now. So you want to make it specific, tangible, actionable, and realistic – not something that’s just vague or squishy, but something that they can actually understand.
The last part of the process is the closing. The purpose of the closing is to transition the audience to your main call to action. Remember, your audience is always asking themselves two questions: “So what?” and “Now what?” And this is where your closing comes in. Your closing is so important because the audience will remember what they learned and heard from you in the final minutes of your talk.
3. Structure your speech
Types of structures for writing your speech.
Another step Baldwin recommended on our podcast on creating your talk is to break your talk into sections beyond the beginning, middle, and end. As you internalize your talk’s message, you can break the talk into sections that you either deliver in order or out of order.
But regardless of how you break it up, you should determine what the point of each section is. It may be to tell a story to illustrate some key thoughts. Practicing that section could include practicing telling the story aloud, delivering the punchline, and transitioning out of that story into the next point that you’re trying to make. This will make it easier to memorize your speech.
Each section should stack on to what you’ve already learned. So once you learn paragraph one, then you can practice paragraph two. Then you can go back and practice one and two together – again, everyone has their own technique, but oftentimes out loud is best! (Another tactic here is to record yourself and listen back to help you to not only learn the material, but to also help decide if the material works.)
Sequential structures
One method Grant Baldwin discusses in our podcast on how to write your speech is to use different types of structures. For example, a sequential structure for memorizing your talk can take the main themes you want to speak about and put them in a sequential form, so that it’s easier to remember the order. Grant gives the example of a talk he gave for college audiences called “Life is a Highway,” where he talked about an imaginary road trip.
As Baldwin said, the way the talk was structured was to talk in the beginning about the past, and where the audience has been, then talk about the future, where they’re going, and to end by talking about where they are, right now. “It needs to almost happen in this certain sequence,” Baldwin said, “which also makes it easier for you to memorize because they need to go in this specific order.”
When you use this structure, you can deliver your speech in any order, Unlike a singer, whose audience may know all the lyrics to the song she sings, if a speaker goes out of order, it may be impossible for the audience to notice – after all, they don’t have a script!
Modular structures
Another type of structure you can use to write your speech is a modular structure. This allows you to go in order, but it also allows you to jump around. This could be especially helpful if you’ve got a couple of main thoughts or ideas and they don’t necessarily have to go in a certain order. You can kind of mix and match them around, similar to how a band at a concert can switch songs around in their setlist.
Baldwin gives the example of topics he covered in a book talk for high school students, answering questions such as, should I go to college? how do I pay for college? What classes do I take? What do I major in? Job interviews, resumes, internships, credit cards, budgets, taxes, etc.
Similar to the sequential structure, it may be helpful for you to think of the content as telling a story, so that you don’t leave anything out. If you have five key themes, for example, that you’d like to cover, they could be five elements of a story you would like to tell. Remember: stories will keep your audience engaged and also make it easier for you to write your speech.
By following these steps, you can set yourself up for success. Many external variables help make a speech go well. Beyond working these steps before giving a speech, you should try to put as many of those variables in your favor as possible. Don’t stay up late the night before at a reception. Don’t eat a massive pasta bowl before you go on stage. Try to avoid speaking during a slot when most of the audience will be distracted. If all the variables are stacked against you but you crush your talk, it can still come across as “meh” to the audience.
Keep in mind: Speaking is like playing jazz – you don’t have to give a talk the same way every time. You can improvise and mix it up sometimes, and you don’t need to plan out every hand gesture or movement or exact line you’ll use. Some of that is fine, but also be present enough with the audience that you can play jazz when the moment calls for it.
If you have a dream to inspire others with your message, you’ve probably considered taking your passion to the stage. Becoming a speaker might sound like a charmed life in many ways. And while it does take hard work, it totally is.
What Type Of Speaker Are You?
Click below to discover your Speaker Archetype and how to start getting booked and paid to speak!
In the meantime, here are a few rapid fire FAQs about speeches. Happy speaking!
How much money can you make as a professional speaker?
The runway to a successful business is often slow. But many professional speakers make 6+ figures a year within a couple years of starting their speaking business!
What degree you need to become a professional speaker?
It does not matter! You can have no degree or a PhD in whatever field you like and still be a great speaker.
Can anyone become a professional speaker?
Absolutely.
How long does it take to become a professional speaker?
This may vary quite a bit, primarily based on your state in life.
- Last Updated: February 29, 2024
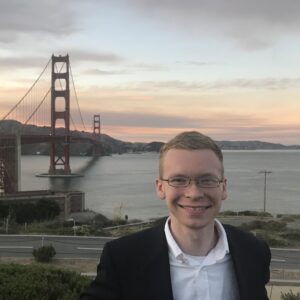
Explore Related Resources
Learn How You Could Get Your First (Or Next) Paid Speaking Gig In 90 Days or Less
We receive thousands of applications every day, but we only work with the top 5% of speakers .
Book a call with our team to get started — you’ll learn why the vast majority of our students get a paid speaking gig within 90 days of finishing our program .
If you’re ready to control your schedule, grow your income, and make an impact in the world – it’s time to take the first step. Book a FREE consulting call and let’s get you Booked and Paid to Speak ® .
About The Speaker Lab
We teach speakers how to consistently get booked and paid to speak. Since 2015, we’ve helped thousands of speakers find clarity, confidence, and a clear path to make an impact.
Get Started
Let's connect.
Copyright ©2023 The Speaker Lab. All rights reserved.
- Bipolar Disorder
- Therapy Center
- When To See a Therapist
- Types of Therapy
- Best Online Therapy
- Best Couples Therapy
- Best Family Therapy
- Managing Stress
- Sleep and Dreaming
- Understanding Emotions
- Self-Improvement
- Healthy Relationships
- Student Resources
- Personality Types
- Guided Meditations
- Verywell Mind Insights
- 2023 Verywell Mind 25
- Mental Health in the Classroom
- Editorial Process
- Meet Our Review Board
- Crisis Support
16 Public Speaking Tips for Students
Arlin Cuncic, MA, is the author of The Anxiety Workbook and founder of the website About Social Anxiety. She has a Master's degree in clinical psychology.
:max_bytes(150000):strip_icc():format(webp)/ArlinCuncic_1000-21af8749d2144aa0b0491c29319591c4.jpg)
Aron Janssen, MD is board certified in child, adolescent, and adult psychiatry and is the vice chair of child and adolescent psychiatry Northwestern University.
:max_bytes(150000):strip_icc():format(webp)/aron-6f9b8a651e804c07b290e57b3218a898.jpeg)
Public speaking tips for students aim to reduce anxiety that can interfere with giving presentations or speeches in class. These tips can also be helpful for those with social anxiety disorder (SAD) who have difficulty speaking in front of a group or telling a story among friends.
Public Speaking Tips
If you have SAD and need to give a speech in elementary school, high school, college, or university, it helps to be as prepared as possible . Beyond preparation, however, there are strategies that you can use to reduce anxiety and fight the urge to stay home with a fake illness.
Even great speakers practice their speeches beforehand. Practice out loud with a recording device or video camera and then watch yourself to see how you can improve. If you are feeling brave, practice in front of a friend or family member and ask for feedback.
- Talk about what you know : If possible, choose a topic for your speech or presentation that you know a lot about and love. Your passion for the topic will be felt by the audience, and you will feel less anxious knowing that you have a lot of experience to draw from when other students ask you questions.
- Concentrate on your message : When you focus on the task at hand, anxiety is less likely to get out of control. Concentrate on the main message of your speech or presentation and make it your goal to deliver that message to the other students in your class.
- Grab the audience's attention : Most of your fellow classmates will pay attention for at least the first 20 seconds; grab their attention during those early moments. Start with an interesting fact or a story that relates to your topic.
- Have one main message : Focus on one central theme and your classmates will learn more. Tie different parts of your talk to the main theme to support your overall message. Trying to cover too much ground can leave other students feeling overwhelmed.
Tell Stories
Stories catch the attention of other students and deliver a message in a more meaningful way than facts and figures. Whenever possible, use a story to illustrate a point in your talk.
Being prepared to speak in public can also be important if you have social anxiety disorder. Feeling confident and prepared to give your speech may help lessen your feelings of anxiety. Some of the things that you can do to prepare include:
- Visit the room : If you have access to the classroom where you will be speaking outside of class hours, take the time to visit in advance and get used to standing at the front of the room. Make arrangements for any audio-visual equipment and practice standing in the exact spot where you will deliver your speech.
- Rack up experience : Volunteer to speak in front of your class as often as possible. Be the first one to raise your hand when a question is asked. Your confidence will grow with every public speaking experience.
- Observe other speakers : Take the time to watch other speakers who are good at what they do. Practice imitating their style and confidence.
- Organize your talk : Every speech should have an introduction, a body, and a conclusion. Structure your talk so that the other students know what to expect.
Manage Your Anxiety
Taking steps to deal with your feelings of anxiety can also make public speaking easier. Some of the things that you can do:
- Tell someone about your anxiety : If you are speaking in front of a high school or college class, meet with your teacher or professor and describe your public speaking fears . If you're in elementary or high school, share your fears with your parents, a teacher, or a guidance counselor. Sometimes sharing how you feel can make it easier to overcome stage fright.
- Visualize confidence : Visualize yourself confidently delivering your speech. Imagine feeling free of anxiety and engaging the students in your class. Although this may seem like a stretch for you now, visualization is a powerful tool for changing the way that you feel. Elite athletes use this strategy to improve performance in competitions.
- Find a friendly face : If you are feeling anxious, find one of your friends in class (or someone who seems friendly) and imagine that you are speaking only to that person.
Press Play for Advice on Finding Courage
Hosted by therapist Amy Morin, LCSW, this episode of The Verywell Mind Podcast shares a strategy to help you find courage when you need it the most.
Follow Now : Apple Podcasts / Spotify / Google Podcasts
Maintain Perspective
Remember that other students are on your side. Think about a time when you have been an audience member and the student delivering the speech or presentation was noticeably nervous. Did you think less of that student? More likely, you felt sympathetic and wanted to make that person more comfortable by smiling or nodding.
Remember—other students generally want you to succeed and feel comfortable. If for some reason the audience is not on your side or you experience bullying or social exclusion, be sure to discuss this with a parent, teacher, or guidance counselor.
Be Confident
Sometimes just knowing what makes a good speech can help you feel more confident. Focus on some of the following elements and practice them before you have to speak in public.
- Develop your own style : In addition to imitating good speakers, work on developing your own personal style as a public speaker. Integrate your own personality into your speaking style and you will feel more comfortable in front of the class. Telling personal stories that tie into your theme are a great way to let other students get to know you better.
- Avoid filler words : Words such as "basically", "well", and "um" don't add anything to your speech. Practice being silent when you feel the urge to use one of these words.
- Vary your tone, volume, and speed : Interesting speakers vary the pitch (high versus low), volume (loud versus soft), and speed (fast versus slow) of their words. Doing so keeps your classmates interested and engaged in what you say.
- Make the audience laugh : Laughter is a great way to relax both you and the other students in your class, and telling jokes can be a great icebreaker at the beginning of a speech. Practice the timing and delivery of your jokes beforehand and ask a friend for feedback. Be sure that they are appropriate for your class before you begin.
- Smile : If all else fails, smile. Your fellow classmates will perceive you like a warm speaker and be more receptive to what you have to say.
Don't Apologize
If you make a mistake, don't offer apologies. Chances are that your classmates didn't notice anyway. Unless you need to correct a fact or figure, there is no point dwelling on errors that probably only you noticed.
If you make a mistake because your hands or shaking, or something similar, try to make light of the situation by saying something like, "I wasn't this nervous when I woke up this morning!" This can help to break the tension of the moment.
A Word From Verywell
It's natural to feel frightened the first time you have to speak in front of your class. However, if you fear continues, interferes with your daily life and keeps you awake at night, it may be helpful to see someone about your anxiety.
Try talking to a parent, teacher, or counselor about how you have been feeling. If that doesn't get you anywhere, ask to make an appointment with your doctor. Severe public speaking anxiety is a true disorder that can improve with treatment .
Spence SH, Rapee RM. The etiology of social anxiety disorder: An evidence-based model . Behav Res Ther. 2016;86:50-67. doi:10.1016/j.brat.2016.06.007
By Arlin Cuncic, MA Arlin Cuncic, MA, is the author of The Anxiety Workbook and founder of the website About Social Anxiety. She has a Master's degree in clinical psychology.
5 Tips on How to Write a Speech Essay
- Homework Tips
- Learning Styles & Skills
- Study Methods
- Time Management
- Private School
- College Admissions
- College Life
- Graduate School
- Business School
- Distance Learning
- M.Ed., Education Administration, University of Georgia
- B.A., History, Armstrong State University
When figuring out how to write a speech, the essay form can offer a good foundation for the process. Just like essays, all speeches have three main sections: the introduction, the body, and the conclusion.
However, unlike essays, speeches must be written to be heard as opposed to being read. You need to write a speech in a way that keeps the attention of an audience and helps paint a mental image at the same time. This means that your speech should contain some color, drama, or humor . It should have “flair.” Make your speech memorable by using attention-grabbing anecdotes and examples.
Determine the Type of Speech You're Writing
Since there are different types of speeches, your attention-grabbing techniques should fit the speech type.
Informative and instructional speeches inform your audience about a topic, event, or area of knowledge. This can be a how-to on podcasting for teens or a historical report on the Underground Railroad. It also can relate to health and beauty, such as "How to Shape Perfect Eyebrows," or hobby-related, such as "Make a Great Bag Out of Old Clothing."
Persuasive speeches attempt to convince or persuade the audience to join one side of an argument. You might write a speech about a life choice, such as, "Abstinence Can Save Your Life," or getting involved in the community, such as "The Benefits of Volunteering."
Entertaining speeches entertain your audience, and topics may not practical. Your speech topic could be something like, "Life Is Like a Dirty Dorm," or "Can Potato Peels Predict the Future?"
Special occasion speeches entertain or inform your audience, like graduation speeches and toasts at celebrations.
Explore the different types of speeches and decide what speech type fits your assignment.
Craft a Creative Speech Introduction
Thoughtco.com / Grace Fleming
The introduction of the informative speech should contain an attention-grabber, followed by a statement about your topic. It should end with a strong transition into your body section.
As an example, consider a template for an informative speech called "African-American Heroines." The length of your speech will depend on the amount of time you have been allotted to speak.
The red section of the speech in the graphic provides the attention-grabber. It makes audience members think about what life would be like without civil rights. The last sentence states directly the purpose of the speech and leads into the speech body, which provides more details.
Determine the Flow of the Body of the Speech
Thoughtco.com / Grace Fleming
The body of your speech can be organized in a number of ways, depending on your topic. Suggested organization patterns include:
- Chronological: Provides the order of events in time;
- Spatial: Gives an overview of physical arrangement or design;
- Topical: Presents information one subject at a time;
- Causal: Shows cause-and-effect pattern.
The speech pattern illustrated in the image in this slide is topical. The body is divided into sections that address different people (different topics). Speeches typically include three sections (topics) in the body. This speech would continue with a third section about Susie King Taylor.
Writing a Memorable Speech Conclusion
The conclusion of your speech should restate the main points you covered in your speech and end with a memorable statement. In the sample in this graphic, the red section restates the overall message you wanted to convey: that the three women you've mentioned had strength and courage, despite the odds they faced.
The quote is an attention-grabber since it is written in colorful language. The blue section ties the entire speech together with a small twist.
Address These Key Objectives
Whatever type of speech you decide to write, find ways to make your words memorable. Those elements include:
- Clever quotes
- Amusing stories with a purpose
- Meaningful transitions
- A good ending
The structure of how to write your speech is just the start. You'll also need to finesse the speech a bit. Start by paying attention to your audience and their interests. Write the words you'll speak with passion and enthusiasm, but you also want your listeners to share that enthusiasm. When writing your attention-grabbing statements, make sure you are writing what will get their attention, not just yours.
Study Famous Speeches
Gain inspiration from others' speeches. Read famous speeches and look at the way they are constructed. Find things that stand out and figure out what makes it interesting. Oftentimes, speechwriters use rhetorical devices to make certain points easy to remember and to emphasize them.
Get to the Point Quickly
Remember to begin and end your speech with something that will gain and hold the attention of your audience. If you spend too much time getting into your speech, people will zone out or start checking their phones. If you get them interested immediately, they will be more likely to stick with you until the end.
Keep It Conversational
How you deliver the speech is also important. When you give the speech , think about the tone you should use, and be sure to write the speech in the same flow that you'd use in conversations. A great way to check this flow is to practice reading it out loud. If you stumble while reading or it feels monotone, look for ways to jazz up the words and improve the flow.
- How to Write and Structure a Persuasive Speech
- How to Write a Narrative Essay or Speech
- 6 Steps to Writing the Perfect Personal Essay
- How to Write a Great Process Essay
- How To Write an Essay
- How to Write a Graduation Speech as Valedictorian
- Tips on How to Write an Argumentative Essay
- What Is Expository Writing?
- Write an Attention-Grabbing Opening Sentence for an Essay
- How to Give an Impromptu Speech
- How to Write a Persuasive Essay
- Understanding Organization in Composition and Speech
- How to Write Your Graduate School Admissions Essay
- Writing a Descriptive Essay
- Writing a Paper about an Environmental Issue
- Memorable Graduation Speech Themes

Speech for Students
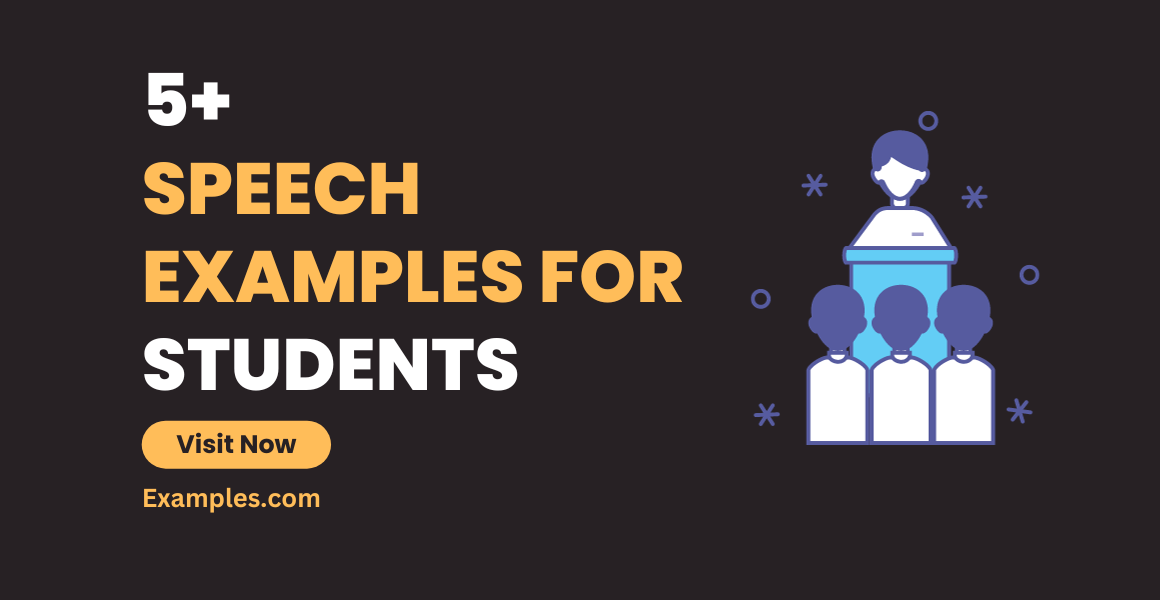
As much as you’ve always excelled in academics, delivering a speech has never been your forte. You just don’t possess the speech skills that some people have. But now you’ve been informed that you’re the class Valedictorian and that you’ll be delivering a simple speech on your graduation day. It can’t be that bad, can it?
Well, you don’t actually have to be the best speech writer to create a good speech. There are various aspects to a good speech in pdf , whether it’s the power brought by your choice of words or you’re ability to stand and deliver a speech.
Student Council Speech
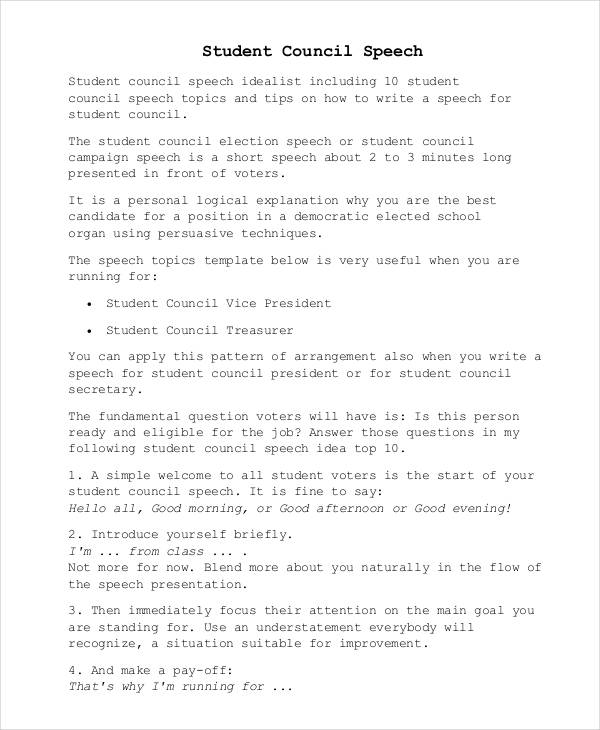
Size: 211 KB
Short Speech for Students
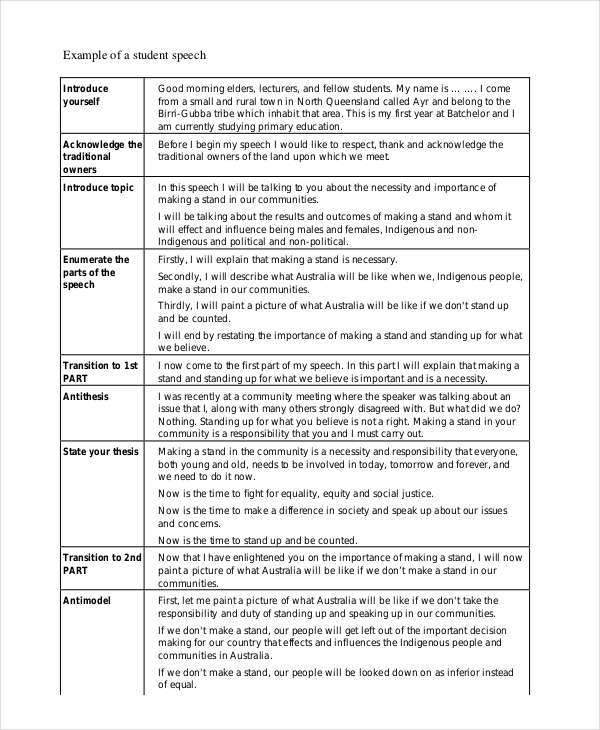
Size: 13 KB
Student Farewell Speech
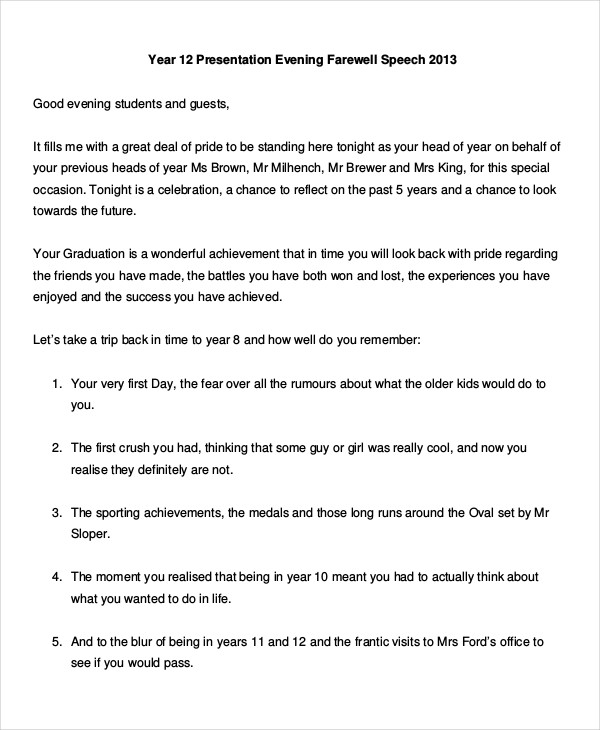
Size: 389 KB
How Is a Student Speech Different from a Regular Speech?
Students are often asked to deliver a speech in front of the class or even the entire student body. This could be to present, inform, or inspire an audience. A student’s speech is usually meant to be straightforward and simple. They aren’t meant to be complex and they follow the standard structure of speech outline templates.
Why Have Student Speeches?
Students are often encouraged to exercise their freedom of speech. It promotes communication and interaction between individuals. This allows them to express their thoughts and protest against actions properly. A speech can help develop a student’s logical reasoning, and it allows them to ponder on various topics and provide insights on what they have learned. It’s also a way for students to continuously gain confidence in themselves. You may also see presentation speech examples & samples.
Furthermore, a student’s speech is often addressed to fellow students and other members of the academe. With this in mind, it allows the speaker to connect with the audience and build a relationship through the speech being delivered. For instance, in an introduction speech , students can be creative with their way of delivering. This is to give meaning and purpose to the speech instead of it seeming as a lecture.
Student Award Example
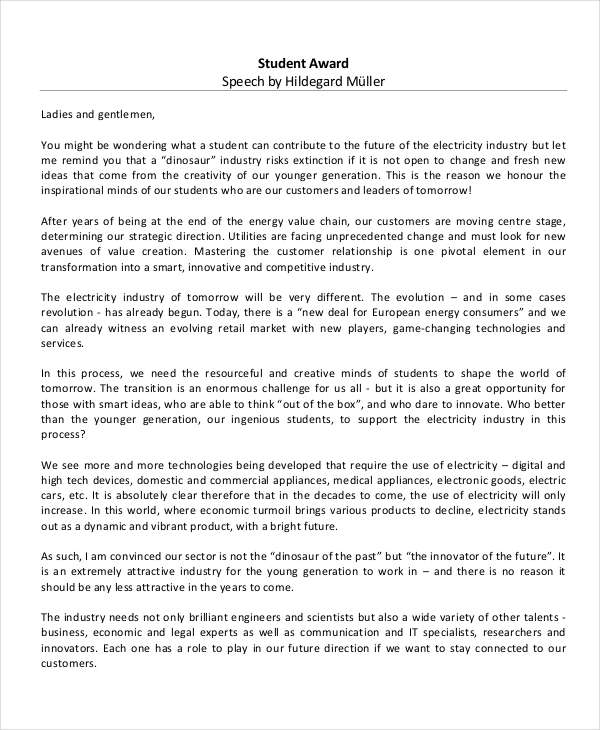
Size: 35 KB
Graduation Speech
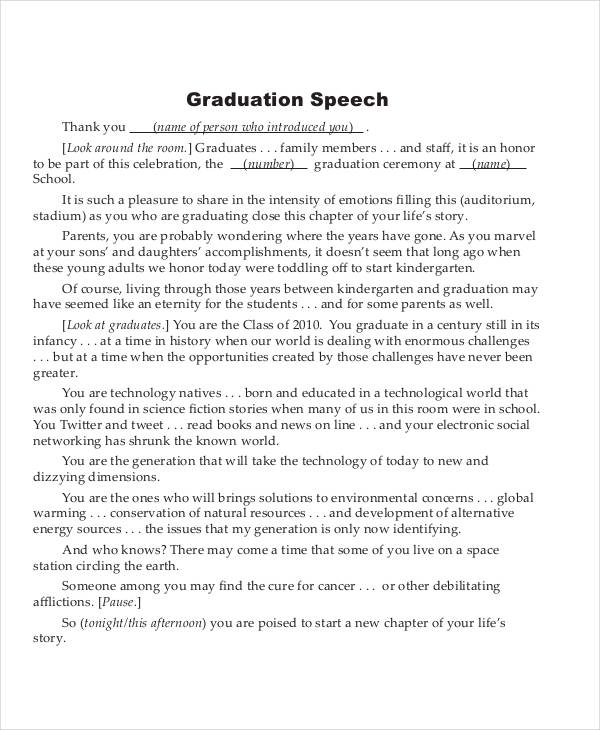
Size: 136 KB
Inspirational Student Example
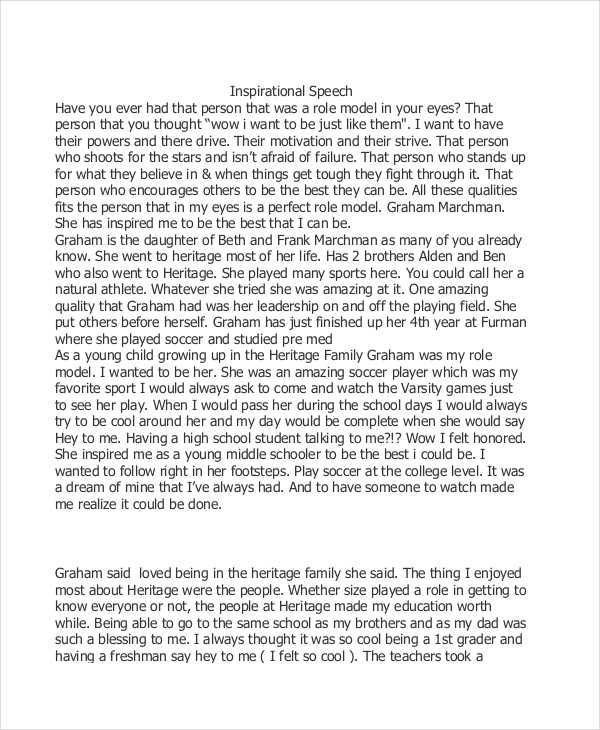
Size: 85 KB
How to Conclude a Student Speech
Student speeches vary in type. Some can be inspiring, such as a graduation speech , while others are simply informative. But creating these types of speeches can be tough. You deliver the speech to a group that you encounter on a daily basis, the type of individuals whose attention span is said to be about 10 to 15 minutes long.
There are various speech examples that tell you how to start a speech but there isn’t much attention given on how to end it. It’s important to keep in mind that your last words will linger in the minds of your listeners making it your last opportunity to leave an impact. Here are a few ways for you to do so:
- Tell a brief story . It should illustrate the main points of your speech.
- Summarize key points . This is to remind your audience of the purpose of your speech. There might have been points that your listeners zoned out on, so it’s good to give them a brief run through of what was covered. You may also like steps in speech composition .
- A call to action . Presenting a challenge to an audience will always leave them thinking. Motivate them with encouraging words and a strong energy.
Speech Generator for Students
Text prompt
- Instructive
- Professional
Create a speech for students on achieving academic success
Help me write a speech for students on the power of perseverance

Speech-writing tips for high school students
by Daniella Dautrich | May 29, 2017 | High school , Teaching Homeschool Writing

Speech-writing Tips for Students
Speech writing offers a rare chance for students to impact an audience in lasting, meaningful ways. Through this kind of written and oral communication, they can learn to convey truth in a world with where morals are blurred and virtues are disappearing. Thus, speech writers combine narrative, descriptive, explanatory, and persuasive skills, arranging a composition to make both logical and emotional appeals . After all, rhetoric (the art of persuasion) should engage the whole person, not just the mind or heart.
Even if your kids will never enroll in a speech and debate club, encourage them to present an original speech in a group setting such as a class, family gathering, or graduation party. These speech-writing tips for students should help them get started!
The Prewriting Stage
When you write a speech, the prewriting stage represents about a third of the entire process.
- Choose a topic you feel strongly about. If you don’t care about the subject matter, neither will your audience.
- Evaluate your potential audience. Will you speak to a mixed group of teenagers or to a room of retirees? What are their values and interests? What kinds of music and cultural references will they relate to?
- Understand your purpose. Are you writing a speech to entertain, inform, or persuade? If you intend to persuade, are you trying to reach a like-minded or neutral audience or an openly hostile group?
- Research and brainstorm. Start gathering your facts and examples, and make a list of possible talking points.
The Writing Stage
Writing the first draft should consume about 20% of your time as a speech writer.
- Develop a “hook.” You need to capture the audience’s attention at the beginning of the speech and motivate them to keep listening. A humorous story or a startling statistic may serve this purpose, depending on the type of speech you’re writing.
- Construct a thesis . Your speech should present a clear message, with each sub-point logically leading to the final conclusion.
- Build a relationship with the audience . Establish your credibility as a speaker by demonstrating your connection to the topic. Did a hobby, a favorite author, or a family experience lead you to choose this subject?
- Organize your ideas . Offer a preview of what’s to come in the introduction, and be sure you follow those points in order.
- Finish with a strong conclusion . When you reach the end of your speech, restate your thesis and tie everything back to your introduction.
The Editing Stage
The editing stage requires another third of your time as a speech writer. As you revise, check for these items:
- Grammar . Poor writing could cause an audience to stop taking you seriously , even if your main message is solid.
- Style. In the writing stage, you focused on substance (what to say); now you can focus on style (how to say it). Without resorting to overdone “ purple prose ,” you can practice writing techniques such as parallelism , repetition, alliteration, and series or lists.
- Time. Read your speech out loud. It shouldn’t take longer than 20 minutes.
- Sound. When you read the speech aloud, do you stumble over unnatural words and phrases? Perhaps you need to rewrite with more direct, simple language. Is your flow of thoughts easy to understand? Is your vocabulary appropriate to the audience’s age and education?
- Appeal to the senses. Your speech should engage the imagination—not put people to sleep! Do you use figurative language to help the audience visualize concepts? Include a descriptive passage to help them hear, feel, and touch your topic. Try to include narratives that people will identify with. You don’t need too many details… just enough to make the stories ring true and help you explain your persuasive points or morals.
- Organization. You can arrange your speech chronologically, topically, by comparison/contrast, or in some other way. Just be sure you’re consistent.
- Politeness . Have you used appropriate language throughout? Have you written with respect for yourself and others? The best speeches display compassion and empathy, rather than tear others down.
The Pre-Performance Stage
Once you’ve written and revised your speech, it’s time to practice! Try to memorize it, and watch your speed so you don’t speak too quickly. Practice in front of a mirror so you remember to move naturally, incorporating hand/arm gestures and facial expressions. Experiment with volume, high and low pitch, and pauses (take notes about what works and what doesn’t.)
Finally, have confidence ! Stage fright is part of life, but the greatest performers have learned that passion and honesty set the speaker—and the audience—at ease every time.
Daniella Dautrich studied classical rhetoric at a liberal arts college in Hillsdale, Michigan.
Let’s Stay Connected!
Subscribe to our newsletter.
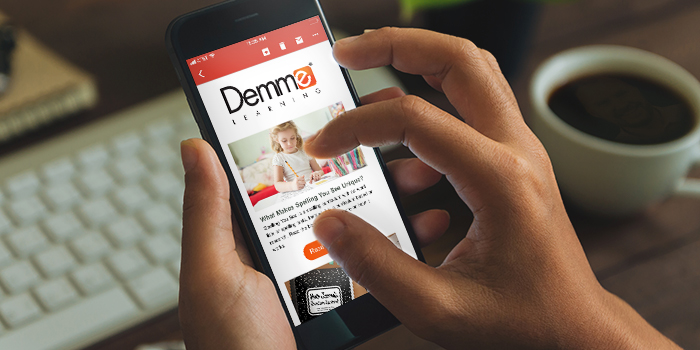
- Gift Guides
- Reluctant or Struggling Writers
- Special Needs Writers
- Brainstorming Help
- Editing & Grading Help
- Encouragement for Moms
- Writing Games & Activities
- Writing for All Subjects
- Essays & Research Papers
- College Prep Writing
- Grammar & Spelling
- Writing Prompts
Recent Posts
- An exciting announcement!
- 10 Stumbling Blocks to Writing in Your Homeschool
- Help kids with learning challenges succeed at homeschool writing
- How to correct writing lessons without criticizing your child
- Games, topic printables & more
- The 4 main speech types
- Example speeches
- Commemorative
- Declamation
- Demonstration
- Informative
Introduction
- Student Council
- Speech topics
- Poems to read aloud
- How to write a speech
- Using props/visual aids
- Acute anxiety help
- Breathing exercises
- Letting go - free e-course
- Using self-hypnosis
- Delivery overview
- 4 modes of delivery
- How to make cue cards
- How to read a speech
- 9 vocal aspects
- Vocal variety
- Diction/articulation
- Pronunciation
- Speaking rate
- How to use pauses
- Eye contact
- Body language
- Voice image
- Voice health
- Public speaking activities and games
- About me/contact
- Speech examples
- Student Council speeches
Student Council Speeches
By: Susan Dugdale
How to write a winning speech: a template, guidelines, plus example speeches
Student Council Speeches mark the end of an election campaign.
Will yours be successful?
The final answer is in the hands of your fellow students. It's entirely their decision.
However, up until they mark their voting papers 'yes' or 'no' you have the potential to make their choice of candidate for the upcoming year 'you'.
How to write a great student council speech
Use the quick links below to find what you need to write a great student council speech, whether it's the President, Vice-President, Secretary or Treasurer role you're after.
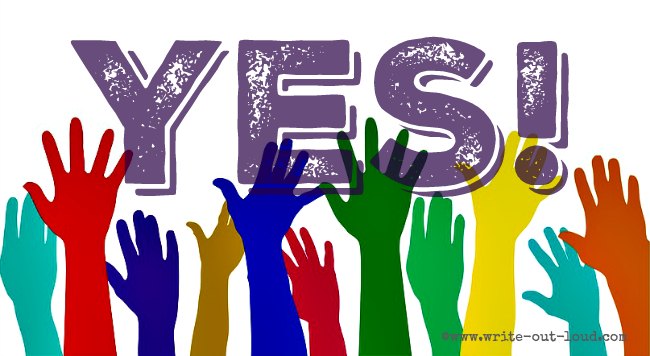
- the primary purpose of your speech
- a template that includes all the necessary elements of a good Student Council speech
- points to consider carefully before you write
- an example Student Council President speech
- an example Student Council Vice President speech
- an example Student Council Secretary speech
- an example Student Council Treasurer speech
- a printable speech planner and outline to download
- vital tips for rehearsal . These make the difference between looking and sounding polished and bumbling.
- a link to a collection of videoed student council speeches
- how to manage anxiety about speaking in front of others
Understanding your speech purpose
Understanding the nature or purpose of your speech could make all the difference between winning and losing.
Student Council speeches are persuasive speeches . Their ultimate goal is to get you the YES vote.
To help you achieve that use the template, (framework or pattern), below to cover all the essential elements you need to pull together.
In addition, it will structure your speech logically, and effectively, from its opening through to its close.
(I've turned the template into a printable enabling you to plan and outline your speech efficiently and easily. You can download it from the link further down the page.)
Return to Top
Student Council speeches template
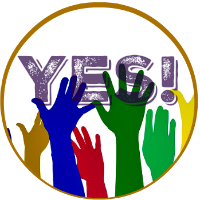
- Greeting - Attention Getter - The Hook You'll need an opening statement or rhetorical question to sit your audience up with open ears and minds. For more see: How to write a speech introduction: 12 of the best ways to start.
- Who you are - your name, your place or grade in the school, and maybe, your hobbies or interests, and the clubs or teams you're a member of. For example, Amnesty International, the speech and debate club, cross-country and basketball. And if you've used a campaign slogan work it in. It'll jog people's memories. 'Ah, yes, that person!', they'll think. Being known and familiar gives you a head start.
- What you want - the role you are campaigning for: President, Vice President, Treasurer, Secretary, Historian...
- What you are going to do for the audience - benefits to them in exchange for their vote. (Brief summary -you will expand this in the body of your speech.)
- Credibility - your qualification or expertise establishing your fitness for the role you want. (Brief summary - you will expand this in the body of your speech.)
- Transition leading to...
- Your Main Idea 1 - For example: your goal for the role, what you want to achieve, how you plan to do it, the benefits to your audience - what painful problem(s) will you solve for them, your fitness for the job, transition to...
- Main Idea 2 - Supporting ideas - details and examples - transition to...
- Main Idea 3 - Supporting ideas - details and examples - transition to...
NB. Only include a second and third idea if you have time to expand on them. If not, move through to the conclusion.
- Summary of main points
- Re-statement of what you want - to be elected to the role you're running for
- Re-statement of the benefits to the audience
- Closer, clincher, call for action
Points to consider BEFORE you write your speech
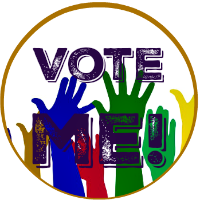
You'll make a better job of completing the printable student council speech template if you take the time to go through the points below.
And then, read the student council speech examples, before you start to write.
Research the role
Think about your audience, what tone or choice of vocabulary is best suited to them.
Avoid trying to impress with either 'big' words or use of slang. Both are traps! Be yourself. Authentic. Real.
Keep your language conversational rather than overly formal and use smaller rather than large sentences.
Try using active rather than passive words. These convey enthusiasm. For examples, see this page on using action verbs . You'll discover how to go from boring bla bla bland to dynamic excitement.
What 'hook' will you use to get them to listen? Humor? Humor is good if it is relevant and inclusive rather than exclusive. (No 'in' jokes!).
Your goal in the role you want
Avoid setting up expectations that you will deliver beyond your capability. :-)
It might be very tempting, but can you really reduce school hours, increase academic standards, introduce a range of exciting new extracurricular activities, as well as have a 'green day' and a movie night every month? Please keep it real!
Your credibility or qualifications
Now is not the time either to be shy or arrogantly big-headed! Let the audience know how right you are for the role you want.
Set yourself apart from other candidates by sharing compelling personal stories or anecdotes that both support your pitch, and show you understand the key issues that matter to your fellow students.
Your school's requirements
If your speech does not meet pre- established criteria in any way you may find it is returned to you edited. It's safer to find out what those criteria are BEFORE writing to avoid having to re-write or worse, being disqualified entirely.
Mockery and personal insults are not clever. They boomerang back on you, letting your audience know you're not to be trusted and neither are you ready for leadership.
Readily acknowledging the skill and expertise of your fellow candidates sincerely in a way that doesn't demean yourself, or them, shows an open mind and maturity.
Aim to have your speech ready BEFORE the deadline.
Give yourself time to prepare thoroughly, including time to review of your opponents' campaigns. That can be very useful for seeing their strengths as well as their weaknesses, which you can then respond to in your own material.
Student Council President speech example
Here's a sample student council speech. I've written it from the perspective of someone running for President.
As you read it, imagine it said aloud. That will help you get the rhythm and flow of language. The speech is between 3 - 4 minutes long, depending on how quickly you speak.
Vote Sophia Clarke for Student Council President
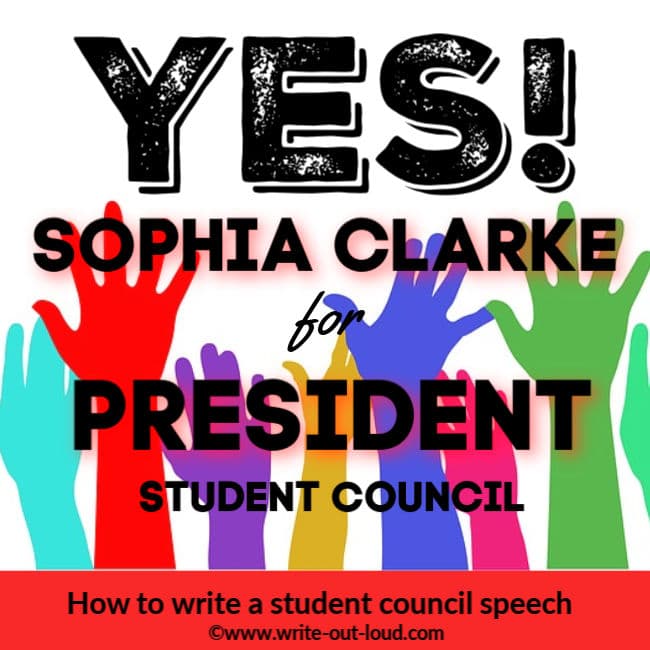
"I’ve got a question for you. I’m not asking you to shout your answer out, or raise your hand. All I’m asking is that you give it room in your mind. Let it sit for a bit, and have a think about it.
My question is – do you believe like I do, that all of us deserve the opportunity to make the best of ourselves? Not second best, 3 rd , or even, highly commended. The BEST.
I’m Sophia Clarke. I’m in the 12 th grade, and I’m running for president. My vision is that each student is enabled to develop the skills and confidence to become the bigger, better version of themselves. The best they can be. Regardless of who they are, and what they need to achieve that.
It’s an audacious goal. Some would say an idealistic, rather than a realistic, one.
However I say it’s awesome. And that you’re intelligent people who realize that reaching any goal starts with taking the first step.
So let me remind you why choosing me, Sophia Clarke, for president, is also choosing a better chance for yourself, and everyone else to grow.
I know you, and I know your needs well. I’ve served on your behalf in multiple roles through my years here; secretary, auditor, public relations officer, and have successfully taken on multiple issues. You’ll know some of those through directly benefiting from them.
It was me who was behind the push to get a regular anti-bullying program running throughout the school. That was two years ago, and now the Teens Against Bullying message underpins what we expect and strive for in our every day dealings with each other.
We know incidents of bullying are far fewer as a result. As our orange tee shirts say we ‘choose kindness, acceptance and inclusion’ for each other, and our selves.
Who has been involved in our mentoring-homework program? Either as a buddy-tutor or as a student getting a helping hand? And who, like me, is passionate about making sure that everybody gets a fair go?
In the past year, under my watch that program has escalated. We have over 50% more tutors across more subject areas and more students taking up the offer of help. That is a fabulous outcome for everybody. Truly win-win.
A tick in the box alongside my name is a tick for the continued growth of those programs. Their value is proven. They allow each of us to grow and experience the strength and confidence that comes from knowing that we can make a positive difference in other people’s lives as well as our own.
When you vote me for President you get my capacity to organize, to liaise, to listen and to speak, working for the benefit of everybody.
A 'yes' for me is a 'yes' for appreciating and celebrating diversity.
A 'yes' for me, Sophia Clarke for President, is 'yes' to a better you.
And together that is a 'yes' to a better life, and a better school, for all of us."
Student Council Vice President speech example
Like the speech above, this one runs to approximately 4 minutes when said aloud. Try it and see.
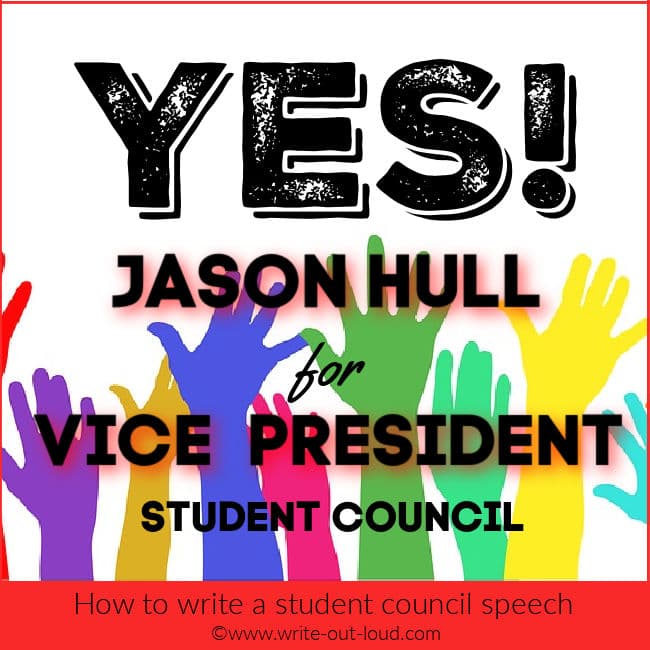
Nod your head if you've heard of the phrase '2nd fiddle' or '2IC'.
What about 'sidekick'?
Not booting a ball in from a sideline but a trusty partner to whoever it is who has the leading role. Like Robin is for Batman.
Or like, {name of your country's Vice President or Prime Minister} is for {name of country's President or Prime Minister} or {name of your school's Vice Principal} is for {name of your school's Principal}!
Well, that's what I aspire to - to become the trusty, tried and true sidekick to the President on our student council.
My name is Jason Hull. I'm in Grade 12 and proudly standing in front of you today as a candidate for the role of Vice President. Yes, I am asking you to give me something of immense value - your vote.
I know what the issues, here at {name of school} are. As part of my campaign, I've interviewed you, and listened. I promise your ideas will be acted on.
Afterall I've trained for this role, put in the time. You know, I know how to get things done.
Last year I served as Secretary and the year before that I was a representative for the committee - proof that I'm committed to bettering our school environment not just for you, but for everybody!
With your support, I'll be your go-to guy when you want to make sure that your opinions and feedback reach the decision-makers.
One of my main goals as your Vice President is to champion your initiatives: amongst others, that's the library extensions you told me about, the desire for healthier food choices in our cafeteria, and the urgent need to increase and diversify the workforce and out-reach opportunities that so many of you mentioned.
Whether you're passionate about improving our school facilities, or enhancing our community involvement, I'll be there to guide and help you.
In the role of Vice President, I will work alongside the President fulfilling my duties to the best of my ability.
Together, we'll make sure that your concerns, and hopes are not just heard but actively pursued. Not 'I' will make sure, but 'we'.
There is no 'I' in we, and that too, is a prerequisite of the Vice President's position: the capacity to put aside ego and to work productively for the good of all.
Because together, we, the Vice President, the President and the other council members, are stronger and can achieve more.
The Vice President role may be a support act but it's a vital one. To succeed in it, collaboration is key. I promise to work hand in hand not only with the President but also with the entire student council team, our teachers, and our administration on your behalf.
Unity is strength. More than ever, we need to nurture understanding, kindness and respect for each other. Regardless of your grade, interests, or background, I want every one of you to feel valued and heard.
That's a goal many would say is impossible.
However, I say, we need to be the difference we want to see in the world. And to borrow those famous words of Helen Keller's: "Alone we can do so little. Together we can so much."
It would be an honor to be your voice, your eyes and your ears as Vice President.
So, I ask you, will you trust me to have your best interests at heart? Will you enable me to work on your behalf?
And are you willing to give me, Jason Hull, your vote for best sidekick, aka. Vice President?
I'll take those smiles, as a 'Yes'.
Example Student Council speeches for Secretary and Treasurer
Click the link to read an:
- example Student Council speech for the role of Secretary . Plus, an overview of the Secretary's main tasks and responsibilities.
- example Student Council speech for the role of Treasurer . Plus, an overview of the Secretary's main tasks and responsibilities.
(This page was getting far too long to include them both here. ☺)
Get the printable student council speech outline
Click on the image below to open a downloadable printable student council speech planner and outline pdf. (Please note it will open in a new window.)
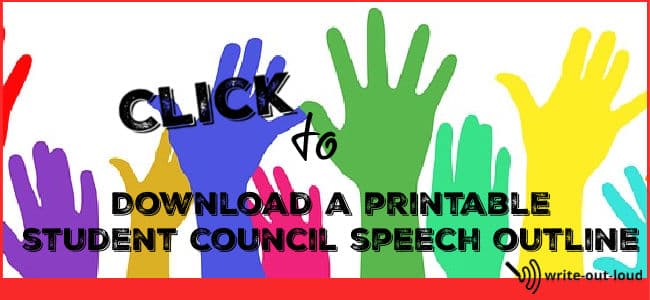
Your completed outline will provide both the structure and the content you need to efficiently write your speech.
After you've finished writing your speech
Now that you've finished writing, you're ready to begin work on your delivery: how you present the speech to your audience.
The first step in that process is making sure your speech fits comfortably into whatever time you've been allocated.
After that comes rehearsal. The information you need for both steps is below.
Timing and word count
Student Council Speeches are generally brief: around 1-4 minutes long which isn't a lot of time! That's between approximately 150 - 600 words at an average speaking rate of 150 words per minute.
To be safe say your speech out loud as if you were delivering it for real and time it. In some schools going overtime can result in being disqualified.
Going faster to fit everything in
Please do not be tempted to say it faster to get everything you planned said. As a strategy it doesn't work. You'll end up gabbling: speaking far too quickly and people won't be able to understand what you're saying.
Cutting out extra material
If you have got too much material for the time limit, cut it. Choose the least important ideas to let go of first. Then move on to rephrasing to reduce the number of words used to express a point.
When you think it's done, repeat the test. Say it out loud as if you were actually giving it, and time it.
If you're now within the allotted time, you are ready for rehearsal.
For more about word count see: how many words per minute in a speech
How to rehearse your speech
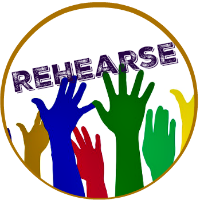
Please, please rehearse your speech ! Do not be tempted to wing it. The more you rehearse the easier it will be to deliver it well.
Remember it is only 1 to 4 minutes long! In that time your goal is to have your audience ready to vote for you.
You can help them make that decision by being confident and prepared. You will show that through:
- your speaking style - natural, sincere, fluent, understandable (clear and able to be heard without straining)
- your body language - relaxed, open gestures, good eye contact and smiling
- your personal grooming or presentation because how you look 'speaks' too. Make sure that your clothing and general grooming supports your speech because, like it or not, you will be judged on both!
Go to: how to rehearse a speech properly .
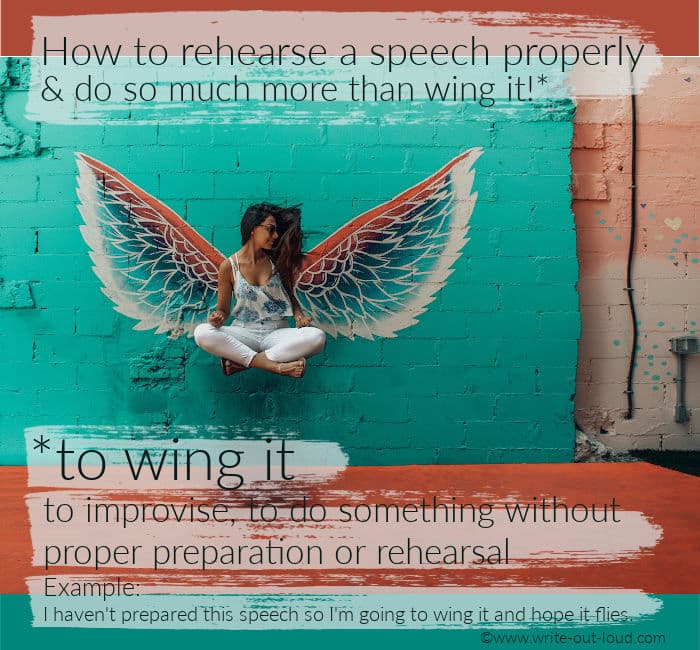
Videoed Student Council speech examples
How do other people handle a Student Council speech? What's their content and delivery like?
Are they funny? Formal? Too hurried? Confident? Familiar with the audience?
It can help to look at what others have done. Even if it's only to decide their way will not be your way!
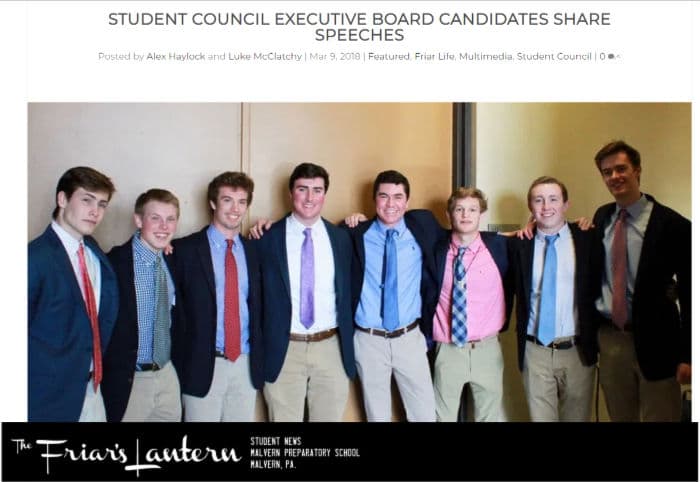
Click the link to access a collection ten videoed student council campaign speeches from the 2018 student council executive board candidates for Malvern Preparatory School, Malvern, Pennsylvania, USA.
At the foot of the article you'll find links to the videos of the school's 2015, 2016 and 2017 student council campaign speeches.
A word of warning
Ps. panic not.
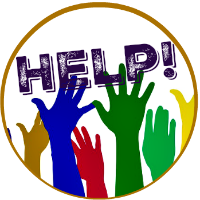
If you find yourself getting anxious over the thought of delivering your speech, please check this page for help.
- How to deal with acute public speaking anxiety: 14 ways that will help
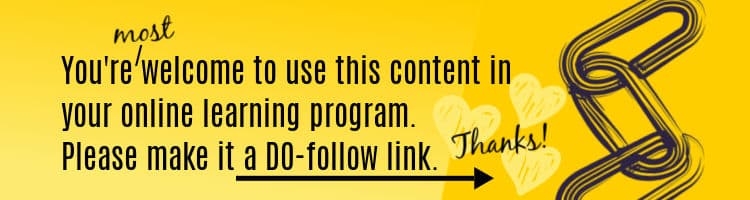
speaking out loud
Subscribe for FREE weekly alerts about what's new For more see speaking out loud
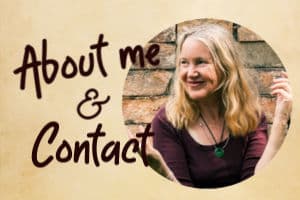
Top 10 popular pages
- Welcome speech
- Demonstration speech topics
- Impromptu speech topic cards
- Thank you quotes
- Impromptu public speaking topics
- Farewell speeches
- Phrases for welcome speeches
- Student council speeches
- Free sample eulogies
From fear to fun in 28 ways
A complete one stop resource to scuttle fear in the best of all possible ways - with laughter.
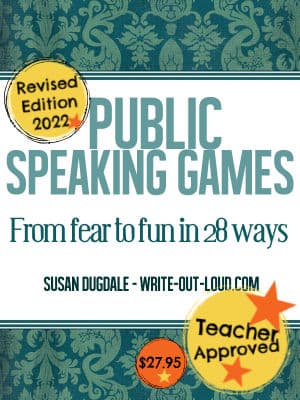
Useful pages
- Search this site
- About me & Contact
- Blogging Aloud
- Free e-course
- Privacy policy
©Copyright 2006-24 www.write-out-loud.com
Designed and built by Clickstream Designs
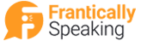
3-minute speeches: Complete guide on writing, preparing and delivering (with examples)
Hrideep barot.
- Body Language & Delivery , Presentation , Public Speaking , Speech Topics , Speech Writing
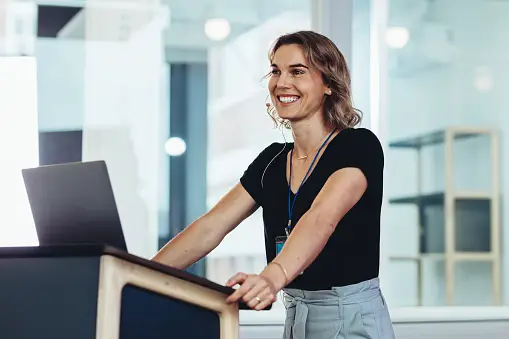
Although 3-minute speeches may seem brief, a lot of words and ideas may be said at that time. Despite the time constraint, if you are conversant with the subject matter, you may prepare for your three-minute speech swiftly. The secret is to create a strong outline that allows you to add or remove details based on how much time you have left.
Words in a 3-minute speech
An average speech of three minutes in length would have roughly 390 words at a regular speech rate of 130 words per minute (wpm) .
Daphne Gray-Grant, a speech and writing coach, discovered that the typical speaking tempo is 125 to 150 words per minute or 375 to 450 words for a three-minute speech .
Read this article for more information: How long should a speech be?
Writing 3-minute speeches
An engaging speech may capture the attention of the crowd and properly capture the spirit of the event. On the other side, if it is poorly written or disorganised, a dozing audience will miss your point. There is no one ideal structure for a speech. You should instead choose what will resonate with your audience the most.
1. Importance of Stories
Beginning with a story engages the audience, and using stories to break up your speech illustrates the concepts you’re talking about using instances from real life. You might also choose to tell snippets of a single story throughout your speech to illustrate your point. If you’re speaking to a group of professional women with kids, for instance, a story about work-life balance is appropriate. Pick a story that is relevant to your audience. Infrequently, if ever, are overtly political or religious statements appropriate.
2. Simply the Facts
It could be advisable to stick to only the facts while presenting a subject while looking for methods to make them applicable to your audience. But no matter what you talk about, keep your speech’s goal in mind at all times. You run the risk of losing the attention of your audience, and making a point with a long, winding speech isn’t particularly effective.
3. Ordered Chronologically
Your tale or the information you’re conveying must make sense in the order you present it, which typically entails using chronological order. If you’re discussing company law, for instance, you should start with earlier laws and tell a tale about how those rules have been modified or updated. It is feasible to deliver a speech in reverse chronological sequence, but you must make sure your audience understands this to avoid misunderstanding. Give the audience frequent dates or other points of reference to serve as the context for the timing of your speech.
4. Organizing Advice
By outlining your speech, you can make sure that you follow the proper flow and concentrate on the most important points rather than getting lost in the weeds of details. Make a rough draught of your speech, then practise it so that it flows naturally before you give it. Take a list of the key points you want to discuss in your speech and consult a subject-matter expert for any information that is either missing or superfluous to ensure that you cover what you need to.
5. Use of Diction
We select our words based on the circumstances we find ourselves in and the audience we are speaking to. The language you would use with your friends might not be appropriate for your boss. Pick the appropriate words for your audience in order to deliver a speech that is effective. The language you use must be clear to them. It is preferable to stay away from using slang because your language must also be acceptable for the topic. You do not need to poke fun at the audience or try to be amusing. Since audiences are able to tell when a speaker isn’t being sincere, speak from the heart and don’t just say what you think the audience wants to hear. You’ll be able to express yourself more strongly and with more emotion as a result.

6. Speech Patterns
Different speeches are needed for different circumstances. A three-minute presentation in class about your ideal job has a different format than a speech to your graduating class. Learning the appropriate format for various speech kinds is simple. There are various varieties of speeches, all of them ranging in form and length. Impromptu, demonstration, educational, persuading, or tribute speeches are a few examples. Each speech is appropriate for a particular situation in life. Select the speech structure that best fits your circumstance, then formats your speech in line with it. Be mindful of your introduction. Strong opening approaches, sometimes known as “hooks,” come in a variety of forms, including tales, rhetorical questions, shocking claims, striking facts, or simply acting in an unexpected or out-of-the-ordinary manner.
Preparing 3-minute speeches
One of the best strategies to make sure you deliver a compelling presentation is to practise your speech beforehand. Consider using these hints to aid in your preparation:
- Your speech should be organised logically with an introduction, body, and conclusion.
- Before giving a speech, frequently practise and rehearse it. Try practising in front of a mirror or with friends acting as your audience. Use a timer to help you pace your speech, and be careful to do so.
- Learn about the podium or other location where the speech will be delivered. Find out the size of the stage, the location of any steps or impediments, and the best places to enter and exit.
- Always keep a professional impression while dressing comfortably .
- Regardless of whether a speech is humorous, serious, or technical, visual aids should fit it. The primary function of visual aids is to facilitate audience comprehension and reinforce key ideas of a speech in distinctive and engaging ways.

Practising 3-minute speeches
It’s common for jittery, distracting body language and a lack of preparation to ruin otherwise effective speeches. Practice your speech after you’ve planned and written it. You have not practised enough if you need to read your speech word-for-word from your notes. You will feel more assured as you practise more. The best way to get rid of tense body language is to have a buddy videotape you speaking so you can see it repeatedly. While speaking, pay attention to how your hands are moving; they should be at ease.
Pay attention to how you stand; you should be tall and straight. Be mindful of your eye contact. Keep your eyes off of your notes, the floor, or the ceiling. Change your jewellery if it is noisy. Don’t touch your face or hair. Do not clasp your hands behind or in front of your back, and refrain from putting your hands in your pockets. Before giving your speech, repeat this procedure multiple times to make sure you have broken these undesirable habits.
The Big Day
Arrive early at the location on the day of your presentation. Wear clean, acceptable apparel, and go for the look that gets you the most compliments. Make sure your cell phone is off and remove any large objects from your pockets. Check your loudness in the room before you start to make sure you are loud enough. Make sure you can make eye contact with those seated at the audience’s margins by paying attention to where they are seated. Check the location in advance to make sure there are no obstacles like wires or cables that could cause you to trip if you wish to move while speaking. All of these actions will support your confidence grows.
Don’t try to improvise during your speech; instead, speak as you did when you were practising. Keep in mind that feeling anxious before, during, and especially after your speech is natural. This is neither a flaw nor a weakness. Use your anxious energy to make your speech lively and engaging if you have done your preparation and practising well.
Delivering 3-minute speeches
Public speaking that is effective and confident should appear natural. In truth, it takes most people a lot of time and practice before they feel comfortable speaking in front of an audience. To overcome stage anxiety, present yourself with confidence, and keep your audience interested, you can employ a variety of tactics.
1. Avoid showing any nervousness while speaking.
Imagine yourself as someone who is imparting knowledge to others who are willing to listen. Don’t worry too much about how you’ll come across while nervous: When a speaker claims to be really frightened, audiences can not help but notice their anxiety. You perceive it as far worse than your listeners do. It doesn’t really matter whether you’re anxious as long as you act calm.
2. Create positive body language
The grin is the most significant facial expression. This forges an immediate connection with your audience and will win them over. Use your facial expressions to emphasise important points: Your speech will look more believable as a result.
It’s important to stand up straight, space your feet slightly apart, and keep your arms at your sides. Do not sway or place your hands on your hips when speaking. You’ll come across as more assured and credible if you appear grounded. Avoid making “closed” motions like crossing your arms or knees or posing for a picture with your arms behind your back. A mental barrier is put up between you and your audience as a result.
3. Use gestures for achievement
To make your message easier for the audience to follow, use your arms and hands. Effective, self-assured body language captures listeners’ attention. Together, your body and words can convey a potent statement. Make sure to vary your gestures to avoid coming out as a robot.
To effectively emphasise ideas, adjust your body language to the size of the area you are working in. Make powerful motions when giving a speech! Tentative, hesitant acts might give you a doubtful, unconvincing appearance.
4. Make eye contact to captivate others.
Make as much eye contact with your audience as you can to engage them (and appear interested in them). Don’t only focus on one welcoming face. Make sure you engage the entire audience by looking at the folks at the rear and on the sides in addition to the front. Observe the crowd more intently than your notes. Not something you read from, notes should be prompt.

How to make a 3-minute speech interesting?
People worry that they will have to “dumb down” their important research due to time constraints, however, this is not the case!
A punchy message and an engaging brief speech can shed light on the breadth of your research and make the worth of your thoughts clear.
If you make the most of your three minutes and plan your speech effectively, you will have plenty of time to accomplish this.
- Deliver a message that is quite obvious.
- Present a “top and tail” component.
- To clarify a complex concept, use metaphors and other verbal illustrations.
- Instead of “making a formal speech,” speak as if you are having a conversation with your listeners.
General topics for 3-minute speeches
3-minute speeches for students.
Everyone has interests, and everyone enjoys discussing them. You know, hobbies could also be passions. Simple inquiries to pose include:
- What interests you?
- Why are your activities so appealing to you?
- When do you engage in these pastimes?
- What are your hobbies, how long have you been doing them, and how did you start?
- What pastimes did you once enjoy but no longer do?
- Is it necessary to engage in hobbies? Why or why not?
Everyone enjoys music, and the majority of people have extremely strong feelings about it, especially when it comes to the music they enjoy (or detest) the most. Simple inquiries to make include the following:
- What genres of music do you enjoy or find boring?
- What emotions do various musical genres evoke in you?
- What genres of music are produced in your nation?
- What song, artist, or album is your favourite?
- What music is now in vogue in your nation?

3. Motivation
Whether or whether the pupils are motivated, it is a good idea to talk about motivation in order to motivate them. Examples of questions are:
- In general, how motivated are you?
- What spurs you on to action?
- What inspires people to accomplish the most?
- What actions do you do when you lack motivation?
- How can one effectively inspire others?
Everybody has objectives, and discussing them really makes us more motivated to take action. Goal-setting can be aided by sharing them with others. An excellent set of inquiries are:
- What are your present life objectives?
- How are your goals going to be attained?
- How frequently do you set yourself goals?
- What objectives have you previously set and attained?
- How do you feel after achieving your objectives?
Everyone has dreams, sometimes on a nightly basis, and discussing them in class is a terrific activity since it encourages pupils to be imaginative and even whimsical. Excellent inquiries on this subject include:
- How would you characterise your dreams?
- What do dreams represent to you?
- What percentage of your dreams can you recall? Why?
- What are your thoughts about prophecies? Are they genuine?
- What are some instances of your most cherished dreams?
Go through this article for more valuable insights: Speech titles and topics: Everything you need to know
Examples of 3-minute speeches
- A 3-minute speech on the topic “Life”.
Good morning to everyone in this room. I’m here today to speak about life and share my opinions with all of you. Life is a never-ending process that must come to an end eventually. Life is all about creating and adoring oneself. a quotation for you: “Life can only be comprehended by living it backwards.” The potential to live a meaningful life and help others do the same is presented by life itself. It makes no difference how long you live. However, how well you lead a good life matter. Death is a constant menace to our life. Everyone will eventually die, but that doesn’t mean we should stop trying to live life to the fullest or pursue our ambitions. A person is only intelligent when they are prepared to face their destiny when it calls, but in the meantime, they should cherish every moment. There is an air of preparation. Every person goes through a journey in life where they must cross the bridge of death in order to awaken to eternal life. Life itself is a genuinely priceless gift. Every moment we have in our life gives us the chance to do something to grow and display our virtues. Every instant unlocks the path for us to accept blessings. The reality is that both good and bad things happen to us in life. What matters most is how we respond. God has given us life as a gift in the hope that we will do our best to make it meaningful. Each of us is a special individual. Respect your uniqueness since no one was born exactly like you and no one ever will be. I frequently come across people who accuse God of something they themselves do not possess. They constantly curse their lives. But do they understand how priceless this life is in and of itself? If we make life worthwhile and strive diligently for positivity. Finally, I’ll say that we ought to make life valuable. Life should be made beautiful by the affection of our family and friends. By carrying out our responsibilities in our families, our workplaces, society, and the larger globe, life can be more beautiful and meaningful.
2. A 3-minute speech by Aaron Beverly who was the 2 nd place winner of the 2016 World Championship of Public Speaking
3. A 3-minute speech by Emma Watson on Gender Equality
Final words
Speaking for three minutes is undoubtedly difficult to master. You must unquestionably conduct an adequate study and choose crucial issues to include in your speech. It is crucial to realise that you must deliver the most essential information first while speaking in a restricted amount of time, such as a 3-minute speech.
A three-minute speech is undoubtedly a wonderful starting point for public speaking. This is because you need to communicate with your audience more effectively when you just have a short amount of time. The speech ought to be concise, pertinent, and clear. Be more relatable to the audience and speak for them. To be the best, you must improve your communication abilities.
Read this article for more useful information: Writing and delivering spectacular short speeches: A-Z guide
Enroll in our transformative 1:1 Coaching Program
Schedule a call with our expert communication coach to know if this program would be the right fit for you
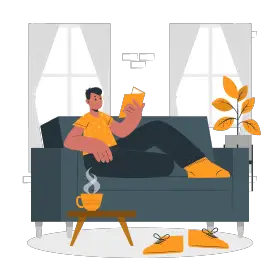
How to Negotiate: The Art of Getting What You Want
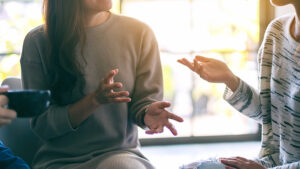
10 Hand Gestures That Will Make You More Confident and Efficient
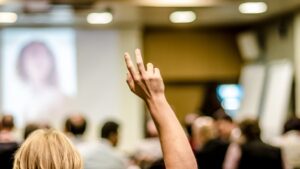
Interrupted while Speaking: 8 Ways to Prevent and Manage Interruptions
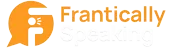
- [email protected]
- +91 98203 57888
Get our latest tips and tricks in your inbox always
Copyright © 2023 Frantically Speaking All rights reserved
Kindly drop your contact details so that we can arrange call back
Select Country Afghanistan Albania Algeria AmericanSamoa Andorra Angola Anguilla Antigua and Barbuda Argentina Armenia Aruba Australia Austria Azerbaijan Bahamas Bahrain Bangladesh Barbados Belarus Belgium Belize Benin Bermuda Bhutan Bosnia and Herzegovina Botswana Brazil British Indian Ocean Territory Bulgaria Burkina Faso Burundi Cambodia Cameroon Canada Cape Verde Cayman Islands Central African Republic Chad Chile China Christmas Island Colombia Comoros Congo Cook Islands Costa Rica Croatia Cuba Cyprus Czech Republic Denmark Djibouti Dominica Dominican Republic Ecuador Egypt El Salvador Equatorial Guinea Eritrea Estonia Ethiopia Faroe Islands Fiji Finland France French Guiana French Polynesia Gabon Gambia Georgia Germany Ghana Gibraltar Greece Greenland Grenada Guadeloupe Guam Guatemala Guinea Guinea-Bissau Guyana Haiti Honduras Hungary Iceland India Indonesia Iraq Ireland Israel Italy Jamaica Japan Jordan Kazakhstan Kenya Kiribati Kuwait Kyrgyzstan Latvia Lebanon Lesotho Liberia Liechtenstein Lithuania Luxembourg Madagascar Malawi Malaysia Maldives Mali Malta Marshall Islands Martinique Mauritania Mauritius Mayotte Mexico Monaco Mongolia Montenegro Montserrat Morocco Myanmar Namibia Nauru Nepal Netherlands Netherlands Antilles New Caledonia New Zealand Nicaragua Niger Nigeria Niue Norfolk Island Northern Mariana Islands Norway Oman Pakistan Palau Panama Papua New Guinea Paraguay Peru Philippines Poland Portugal Puerto Rico Qatar Romania Rwanda Samoa San Marino Saudi Arabia Senegal Serbia Seychelles Sierra Leone Singapore Slovakia Slovenia Solomon Islands South Africa South Georgia and the South Sandwich Islands Spain Sri Lanka Sudan Suriname Swaziland Sweden Switzerland Tajikistan Thailand Togo Tokelau Tonga Trinidad and Tobago Tunisia Turkey Turkmenistan Turks and Caicos Islands Tuvalu Uganda Ukraine United Arab Emirates United Kingdom United States Uruguay Uzbekistan Vanuatu Wallis and Futuna Yemen Zambia Zimbabwe land Islands Antarctica Bolivia, Plurinational State of Brunei Darussalam Cocos (Keeling) Islands Congo, The Democratic Republic of the Cote d'Ivoire Falkland Islands (Malvinas) Guernsey Holy See (Vatican City State) Hong Kong Iran, Islamic Republic of Isle of Man Jersey Korea, Democratic People's Republic of Korea, Republic of Lao People's Democratic Republic Libyan Arab Jamahiriya Macao Macedonia, The Former Yugoslav Republic of Micronesia, Federated States of Moldova, Republic of Mozambique Palestinian Territory, Occupied Pitcairn Réunion Russia Saint Barthélemy Saint Helena, Ascension and Tristan Da Cunha Saint Kitts and Nevis Saint Lucia Saint Martin Saint Pierre and Miquelon Saint Vincent and the Grenadines Sao Tome and Principe Somalia Svalbard and Jan Mayen Syrian Arab Republic Taiwan, Province of China Tanzania, United Republic of Timor-Leste Venezuela, Bolivarian Republic of Viet Nam Virgin Islands, British Virgin Islands, U.S.
- Delivery Techniques →
Student Council Speech Ideas: How to Craft the Perfect Speech
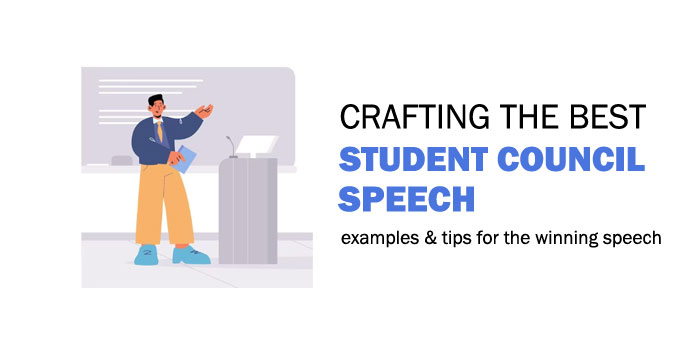
Now that you’ve decided to take on the challenge of running for student council, it’s time to consider how to craft that all-important speech that will make your candidacy stand out from the rest.
It goes without saying that writing the perfect speech requires some preparation and a variety of creative approaches. This post will provide you with student council speech ideas that will help you put together a memorable and persuasive talk that will propel your candidacy.
Here, we will look at ways to structure your speech, as well as techniques for convincing the student body that you’re the right person for the job. With the following tips, you’ll have the winning speech that gets you elected!
Understand the Position and Responsibilities
When crafting the perfect student council speech, it is important to understand the position and responsibilities associated with a student council leader.
Student council leaders are elected by fellow students to represent their class or school in governance-related decisions. In addition to seeking input from peers, they often work with faculty members, administrators, and other key stakeholders when making major decisions.
Being a student council leader requires strong interpersonal skills and the ability to think critically. It is important to understand the roles and responsibilities of other student leaders, staff, and faculty members alike so that collaboration can be fostered under one’s leadership.
Not only is it essential to maintain positive relationships but also to demonstrate proficiency in areas such as problem solving, communication, and decision-making.
While some may view the role of a student council leader as administrative paperwork and organizational tasks, a leader should have an understanding of more than just policymaking. They must be able to promote good citizenship among peers and create an atmosphere that encourages engagement.
Drafting the perfect student council speech should not be done without regard for the objectives of a student council leader.
When speaking on behalf of peers it is essential to remember that both knowledge of positions and values are required for successful leadership. With this in mind we can move forward into the next section where we explore “What Does a Student Council Leader Do?”
What Does a Student Council Leader Do?
Being a student council leader requires more than just giving a speech .
The responsibilities of leading the student council consist of building productive relationships, setting ambitious goals and executing plans, communicating effectively, listening to peers and understanding their concerns, advocating for students’ rights in the school and community, and organizing events to benefit the student body.
Leaders must possess both soft skills such as being organized and hard skills like public speaking . With both sets of skills, these leaders are able to skillfully manage a team or individual volunteers and create a focus on important goals that the council wants to accomplish.
A successful student council leader should have a strong sense of responsibility and accountability while also displaying enthusiasm towards achieving collective goals.
The debate can be seen as to whether or not student council leaders should participate more in decision-making power or serve more as figureheads for their group goals.
Advocates for more decision-making authority argue that student councils can be valuable partners with school administrative teams by providing insight into issues that face students directly and providing perspective into how policies are playing out in the student body.
Opposing sides argue that although student councils can provide insight into certain issues, they cannot be expected to make major decisions regarding school policies given their lack of experience with those matters.
No matter the opinion on this debate, all agree that student council leaders play an integral role in school culture and environment by representing their peers. Those seeking election to a leadership role must demonstrate their commitment to furthering the mission of their student body.
This is done through crafting speeches that elucidate why they’re qualified for the position as well as show evidence of previous service accomplishments. To learn how to do this properly, it is useful to understand how to craft the perfect speech by demonstrating your experience.
Demonstrate Your Experience
When crafting your student council speech, it is important to demonstrate your experience and expertise.
Being able to speak confidently about your qualifications and previous accomplishments will help convince your audience of your ability to take on a leadership role.
Start off with a statement that introduces yourself and the reasons why you are qualified for the position. Use specific examples of projects or responsibilities that you have undertaken in the past, as well as how you were successful in completing them.
You may want to describe any volunteer or organizational activities that you are involved in or any educational qualifications or awards that you may have earned.
It is also worthwhile debating the various pros and cons of your candidacy. This will demonstrate your ability to think analytically, as well as showcase your knowledge on the subject matter. However, be warned to not make any personal attacks or remarks, which could alienate portions of your audience if they disagree with your viewpoint.
The next section will discuss how to talk about your leadership skills and convince your audience of their importance in making an impactful student council member.
Most Important Summary Points
When crafting a student council speech, it is important to demonstrate experience and qualifications to ensure an effective appeal to the audience. Talking about past achievements and providing specific examples will help make a case for why you are the best fit for the position.
Additionally, it may be beneficial to provide an analytical debate stating both the pros and cons of your candidacy; however, it should be done without making any personal attacks on opponents. Finally, discussing leadership skills and their relevance to the role may also work in favor of your candidacy.
Talk About Your Leadership Skills
When crafting a student council speech, it is important to talk about one’s qualifications in order to demonstrate leadership.
Before doing so, it is beneficial to list tangible accomplishments, such as working on a particular project or volunteering for an organization. It is also important to discuss any awards won or leadership roles held prior to speaking in front of the student council.
Being able to communicate one’s importance and impact helps prove why one is the best candidate for the role and can potentially sway the audience in their favor.
On the other hand, discussing past and current leadership roles does not always need to include tangible accomplishments.
Talking about oneself in terms of what they have learned and how they plan on using it moving forward can also be very powerful. For example, talking about mistakes made, lessons learned, and how one plans on incorporating that knowledge into their candidacy can show wisdom beyond their years.
It can also serve as proof that even though they may be young, they are still capable of making a great contribution to the student council if elected.
Ultimately, striking a balance between tangible accomplishments and experiences learned will ensure that your leadership speaks for itself during your student council speech.
By combining both sides of the argument and effectively conveying them to your audience, you will be best positioned for success when discussing your leadership skills with potential voting members of the student council. Now, let’s move on to discussing our ideas and goals for being on the council if given the opportunity.
Discuss Your Ideas and Goals
When discussing your ideas and goals, it is important to be direct and succinct. Start by introducing yourself and briefly outlining why you are running for Student Council in the first place.
Explain what inspired you to run and what you hope to bring to the table. Being clear and concise while still providing tangible examples will help voters better understand both your dedication and drive.
Once your motivation has been made clear, detail the specific things you plan to change or implement as a representative of the student body if elected.
Speak from the heart and talk about what issues are most important to you. During this time, it is crucial that you have done research on the particular problems that may be faced by the student council at their respective school. Then, use this information to inform your ideas, goals, and propositions on any particular issue.
In addition to thoroughly preparing for your speech in advance, it is also a good idea to practice beforehand. Students should train themselves not only in speaking but also in presenting facts throughout speech so that they can defend their ideas if someone questions them.
To ensure success during a presentation, test yourself with hard questions, rehearse extensively and get comfortable with the material ahead of time.
Lastly, students should remember that their goal is to deliver an effective speech which can influence enough people’s desire to make a difference within their school and community.
Debating both sides when discussing your ideas and goals is a great way to both show your understanding of a particular subject and present yourself as an engaged student looking to truly make a change.
Showing awareness of how opposing views affect policy changes can demonstrate your analytical skills while making sure everyone hears all sides of an argument allows for more informed decision-making among fellow students.
With this understanding in place, students can then start considering proposed changes or improvements for their respective student councils with confidence.
Explain Proposed Changes and Improvements
When proposing changes and improvements in a student council speech, it is important to explain why the proposed changes are necessary.
In order to effectively discuss both sides of the argument, craft your speech so that you first suggest why the changes are needed, followed by possible counterarguments and how you plan on addressing them.
Explain the current problems and deficiencies within your community or organization, as this can help emphasize why the suggested changes are needed.
For example, if you are suggesting increasing funding for student sports teams, you could draw attention to their lack of proper equipment and uniforms due to not enough money.
Offering solutions on how to acquire more funds is also important; brainstorm with other student council members to come up with ideas like requesting donations from teachers or local businesses.
In addition, anticipate and address any doubts that your peers may have about the suggested changes. Be aware of other perspectives surrounding the issue at hand and discuss every angle in detail in your speech.
If some students feel as though certain aspects of the changes will cost too much for their comfort, propose ways that costs can be kept low without sacrificing results.
Additionally, point out positive aspects of the proposed changes from multiple points of view, such as how they benefit various groups within the school or organization.
Explaining proposed changes and improvements is a key tool in crafting an effective student council speech. It’s important to detail why each change is necessary and offer solutions on how to make it happen while also standing firm on your viewpoint.
By showing dedication and commitment towards these ideas through a comprehensive analysis of both sides of each argument , you’ll be able to give an impactful speech that resonates with your peers.
Show Dedication and Commitment
When crafting a student council speech, showing dedication and commitment is key. This is important to discuss with students as they prepare to give their speeches because it will help them to sound confident in their ability to carry out the tasks of being on student council.
This can be done through highlighting past accomplishments that demonstrate a commitment to projects, as well as pledging to continue to work hard on future initiatives. It is also beneficial for speakers to emphasize the importance of making an impact both on campus and within the broader community.
It is important to highlight both sides of the argument when discussing dedication and commitment. For example, it can also be beneficial to discuss how there are many challenges in achieving objectives, but if one is motivated and determined, goals can still be realized.
Also, considering how student council represents a wide array of points of view and perspectives, it is essential for speakers to commit to being open-minded when discussing issues. Acknowledging opposing views with respect shows dedication and commitment not only to staying civil but also representing everyone in student council effectively.
Ultimately, dedicating time, energy and effort and remaining committed throughout this process is essential for success when it comes to executing student council duties.
By showcasing these qualities during a speech, speakers have the opportunity to demonstrate determination and initiative that fellow students should admire and stand behind. With that in mind, addressing the student body appropriately will help ensure an effective presentation overall.
Address the Student Body Appropriately
When addressing the student body, an important consideration for any Student Council speech should be to consider the audience.
After all, this is ultimately who the message is intended for. You want to make sure that you craft a message that speaks to and resonates with your peers.
This can be as simple as mentioning a common interest or having a connection with something they can relate to, such as pop culture.
However, it is also important to remember to maintain a certain level of professionalism in your speech throughout so as not to alienate members of the student body or allow the tone and atmosphere of the occasion to become unwelcoming.
It is also important to address different members including teachers involved in helping put together the council, staff members and other bodies involved in school activities.
It can also be beneficial to openly thank those involved for their hard work and dedication regardless of opinion or stance during preparations for the council meeting and election process.
This shows respect from one side as well as appreciation from you—to endear yourself more towards the wider student body.
Regardless of the approach when addressing fellow students, no matter how persuasive or heartfelt they may be, you will want to make sure that your words are not viewed as inflammatory or disrespectful.
Consideration must be taken into account when making statements that highlight potential issues within a school or resident authority because these can cast a negative light on you as well as them.
A balanced approach where both sides are heard endorses fairness towards each viewpoint and encourages healthy debate among members of the student body.
Having established what’s appropriate when talking directly to fellow students, it is now time to explore ways of creating a powerful speech to ensure that your message hits all the right notes. The next section will discuss ways in which you can prepare a speech that has maximum impact on the student body.
Prepare a Speech that Hits All the Right Notes
Preparing a speech for student council can be an intimidating process. It is important to make sure that you hit all the right notes within your speech. Through careful planning, practice and consideration of your audience, it is possible to construct an impactful, memorable speech.
When writing your speech, begin by recognizing the qualities that make you a great candidate. Detail the goals of your platform by articulating the various ways in which you will serve your constituents and their needs.
Demonstrate a clear understanding of the real issues facing students and illustrate how you understand their perspectives on them. Showcase your character by outlining concrete actions that demonstrate why you are uniquely qualified for the position.
The most important part of any speech is having a strong conclusion . Your conclusion should restate the core message and summarize some of the key points from throughout the entirety of the address.
Additionally, include a call to action that encourages students to get involved – whether simply by voting or actively campaigning on behalf of your candidacy.
Make sure to end on an inspiring note that reinforces your leadership skills and offers an optimistic outlook for the future of the student body with you as their representative.
Closing Remarks and Final Thoughts on Your Student Council Speech
When crafting your closing remarks, the most important thing to remember is to keep it brief and to the point. Try not to repeat yourself and instead emphasize key points that you want your audience to remember. Here are some tips on how to close your student council speech with impact:
1. Summarize Your Ideas: After spending time talking about your ideas and plans, briefly summarize your message in a few sentences. If you have raised any specific issues, make sure to include any solutions or opinions that you have presented in the course of your speech.
2. Express Gratitude: Show your appreciation towards all those who voted for you and stuck around to listen, thanking them for their time and energy spent affirming their belief in you as a capable leader.
3. Leave On A High Note: End on a high note by expressing optimism about the future outcomes of the initiatives you raised during your speech, no matter what the results may be. Take this opportunity to give the audience a feeling of immense hope and excitement, ensuring they depart with a positive outlook on all that can be achieved through leadership.
4. Keep It Short And Simple: Above all, ensure that whatever final words you choose express confidence in yourself and remember that overthinking can derail your performance. Do not give too much information but rather provide powerful points that will ensure everyone knows where you stand—then step down!
In conclusion, taking into consideration the tips outlined above when creating your closing remarks will ensure dramatic effect when delivering a successful student council speech. Keep it short, sweet, memorable and sincere—the perfect recipe for an effective finish!
Frequently Asked Questions
What are the most important elements to include in a student council speech.
One of the most important elements to include in a student council speech is the audience. When crafting the perfect speech, it is important for the speaker to consider who their audience is, and how their topic will be received. It’s best to tailor the content to fit the interests of the particular audience, so that they can relate more directly to what you’re saying.
Another key element would be credibility. The speaker should provide solid evidence and facts behind their ideas, in order to gain trust from their audience and prove that their points are valid and reliable. This includes anticipating any questions or counterarguments and being prepared with justifications for any potential criticism.
Additionally, the organization of the speech should be a priority. The speaker should have an outline or plan in place beforehand, so as not to get lost or overwhelmed in the middle of their address. Having an organized structure helps maintain unity throughout the speech, while promoting a professional presentation overall.
Finally, an effective student council speech should also tell a story . Incorporating personal anecdotes or experiences related to your topic can help make your words more memorable and meaningful to your audience. Doing this can also create a more intimate connection between yourself and whoever is listening.
How should a student council speech be structured?
A student council speech should be structured in such a way as to effectively communicate the speaker’s ideas while keeping their audience engaged .
The speech should generally start with an attention-grabbing introduction , followed by a statement of purpose or goal that outlines the speaker’s main argument. The body of the speech should then provide evidence and reasoning for why the argument is valid. Finally, the conclusion of the speech should reiterate the key points, summarize the overall message and close with a memorable quote or call to action.
In order for a student council speech to be effective, it must be well organized and cohesive. To ensure this, each point should be supported by clear examples and logical evidence .
Additionally, arguments and information should be presented in chronological order, making it easy for listeners to follow the flow of thought being presented. Visual aids can also be extremely helpful in breaking up sections of longer speeches while providing supporting images and facts.
Overall, crafting the perfect student council speech involves organizing information into an understandable format while creating an engaging story throughout. By highlighting key arguments and providing persuasive evidence along the way, presenters have a better chance of having their message heard and remembered.
What are some effective techniques for delivering a student council speech?
When delivering a student council speech, there are a few key techniques that can help make it more effective.
1. A Clear Starting Point: Start your speech by introducing yourself and briefly explain why you are running for office. Make sure to be extremely clear with your audience so they understand where you stand.
2. Speak Intentionally: Speak slowly and intentionally so your points come across clearly without stuttering or stumbling over words. Pause in order to better make your point, gather your thoughts, and allow the audience to digest what you’ve said.
3. Use Audience Engagement: Ask the audience questions and provide them with opportunities to show their support or contribute to the conversation. This is an effective way of getting the audience involved while also allowing you to gauge their interests or lack thereof, thus providing feedback that can inform your future decisions if elected.
4. Establish Goals: Provide realistic and achievable goals that you plan to accomplish during your time in office, should you be elected. Showing a desire for change and progress will ensure that the audience understands what you are striving for and hopefully motivate them to support you in achieving it.
5. Rehearse: Rehearase tiyour speech multiple times beforehand in front of friends, family members, or even teachers, in order to become comfortable with every aspect of it – from its content to its delivery – as this helps create an easier flow throughout your delivery when done live.
Above all else, it is important that you remain confident in what you are saying and who you are as a person – this will give the audience assurance that they are voting for the right candidate who is able to fulfill their goals and expectations as student council president!
Speech Writing
Speech Examples

20+ Outstanding Speech Examples for Your Help
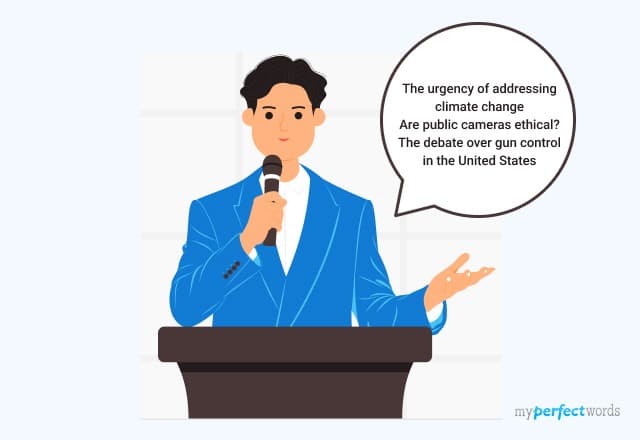
People also read
The 10 Key Steps for Perfect Speech Writing
Understanding the Speech Format - Detailed Guide & Examples
How to Start A Speech - 13 Interesting Ideas & Examples
Common Types of Speeches that Every Speechwriter Should Know
Good Impromptu Speech Topics for Students
Entertaining Speech Topics for Your Next Debate
How to Write a Special Occasion Speech: Types, Tips, and Examples
Introduction Speech - A Step-by-Step Guide & Examples
How to Write the Best Acceptance Speech for Your Audience?
Presentation Speech - An Ultimate Writing Guide
Commemorative Speech - Writing Guide, Outline & Examples
Farewell Speech - Writing Tips & Examples
How to Write an Extemporaneous Speech? A Step-by-Step Guide
Crafting the Perfect Graduation Speech: A Guide with Examples
Public speaking can be daunting for students. They often struggle to start, engage the audience, and be memorable. It's a fear of forgetting words or losing the audience's interest.
This leads to anxiety and self-doubt. Students wonder, "Am I boring them? Will they remember what I say? How can I make my speech better?"
The solution lies in speech examples. In this guide, we'll explore these examples to help students create captivating and memorable speeches with confidence.
So, keep reading to find helpful examples!

- 1. Speech Examples
- 2. Tips to Write a Good Speech
Speech Examples
Talking in front of a bunch of audiences is not as easy as it seems. But, if you have some good content to deliver or share with the audience, the confidence comes naturally.
Before you start writing your speech, it is a good idea that you go through some good speech samples. The samples will help to learn how to start the speech and put information into a proper structure.
Speech Examples for Students
Speech writing is a huge part of academic life. These types of writing help enhance the creative writing skills of students.
Here is an amazing farewell speech sample for students to learn how to write an amazing speech that will captivate the audience.
Below, you will find other downloadable PDF samples.
Speech Examples for Students
Every school and college has a student council. And every year, students elect themselves to be a part of the student council. It is mandatory to impress the student audience to get their votes. And for that, the candidate has to give an impressive speech.
Here are some speech examples pdf for students.
Speech Examples For Public Speaking
Speech Examples About Yourself
Speech Examples Short
Speech Examples For College Students
Speech For Student Council
Speech Examples Introduction
Speech Example For School
Persuasive Speech Examples
The main purpose of a speech is to persuade the audience or convince them of what you say. And when it comes to persuasive speech , the sole purpose of speech becomes more specific.
Persuasive Speech Example
Informative Speech Examples
Informative speeches are intended to inform the audience. These types of speeches are designed to provide a detailed description of the chosen topic.
Below we have provided samples of informative speech for you.
Informative Speech Example
Informative Speech Sample
Entertainment Speech Examples
Entertainment speeches are meant to entertain the audience. These types of speeches are funny, as well as interesting. The given speech samples will help you in writing an entertaining speech.
Entertainment Speech Example
Entertainment Speech Sample
Argumentative Speech Examples
Making a strong argument that is capable of convincing others is always difficult. And, when it comes to making a claim in an argumentative speech, it becomes more difficult.
Check out the argumentative speech sample that demonstrates explicitly how an argumentative speech needs to be written.
Argumentative Speech Example
Demonstration Speech Examples
The demonstrative speeches are intended to demonstrate or describe the speech topic in depth. Get inspired by the demonstrative speech sample given below and write a captivating demonstrative speech.
Demonstration Speech Example
Demonstration Speech Sample
Motivational Speech Examples
Motivational speeches are designed to motivate the audience to do something. Read out the sample motivational speech given below and learn the art of motivational speech writing.
Impromptu Speech Examples
Impromptu speech writing makes you nervous as you are not good at planning and organization?
Check out the sample impromptu speech and learn to make bullet points of your thoughts and plan your speech properly.
Graduation Speech Examples
Are you graduating soon and need to write a graduation farewell speech?
Below is a sample graduation speech for your help.
Wedding Speech Examples
“My best friend’s wedding is next week, and I’m the maid of honor. She asked me to give the maid of honor speech, but I’m not good at expressing emotions. I’m really stressed. I don’t know what to do.”
If you are one of these kinds of people who feel the same way, this sample is for you. Read the example given below and take help from it to write a special maid of honor speech.
Best Man Speech Examples
Father of The Bride Speech Example
Speech Essay Example
A speech essay is a type of essay that you write before writing a proper speech. It helps in organizing thoughts and information.
Here is a sample of speech essays for you to understand the difference between speech format and speech essay format.
Tips to Write a Good Speech
Reading some famous and incredible sample speeches before writing your own speech is really a good idea. The other way to write an impressive speech is to follow the basic tips given by professional writers.
- Audience Analysis: Understand your audience's interests, knowledge, and expectations. Tailor your speech to resonate with them.
- Clear Purpose: Define a clear and concise purpose for your speech. Ensure your audience knows what to expect right from the beginning.
- Engaging Opening: Start with a captivating hook – a story, question, quote, or surprising fact to grab your audience's attention.
- Main Message: Identify and convey your main message or thesis throughout your speech.
- Logical Structure: Organize your speech with a clear structure, including an introduction, body, and conclusion.
- Transitions: Use smooth transitions to guide your audience through different parts of your speech.
- Conversational Tone: Use simple, conversational language to make your speech accessible to everyone.
- Timing: Respect the allocated time and write the speech accordingly. An overly long or short speech can diminish the audience's engagement.
- Emotional Connection: Use storytelling and relatable examples to evoke emotions and connect with your audience.
- Call to Action (if appropriate): Encourage your audience to take action, change their thinking, or ponder new ideas.
- Practice Natural Pace: Speak at a natural pace, avoiding rushing or speaking too slowly.
So, now you know that effective communication is a powerful tool that allows you to inform, persuade, and inspire your audience. Throughout this blog, we've provided you with numerous examples and invaluable tips to help you craft a compelling speech.
And for those moments when you require a professionally written speech that truly stands out, remember that our team is here to help. We can rescue you from writer's block and deliver an outstanding speech whenever you need it.
With our essay writing service , you can be confident in your ability to communicate your message effectively and leave a lasting impact.
So, don't hesitate – place order now and buy speech that will truly captivate your audience.

Write Essay Within 60 Seconds!

Dr. Barbara is a highly experienced writer and author who holds a Ph.D. degree in public health from an Ivy League school. She has worked in the medical field for many years, conducting extensive research on various health topics. Her writing has been featured in several top-tier publications.

Paper Due? Why Suffer? That’s our Job!
Keep reading
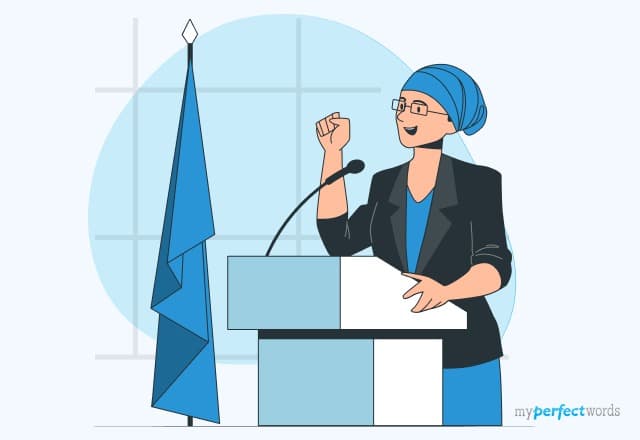
- PRO Courses Guides New Tech Help Pro Expert Videos About wikiHow Pro Upgrade Sign In
- EDIT Edit this Article
- EXPLORE Tech Help Pro About Us Random Article Quizzes Request a New Article Community Dashboard This Or That Game Popular Categories Arts and Entertainment Artwork Books Movies Computers and Electronics Computers Phone Skills Technology Hacks Health Men's Health Mental Health Women's Health Relationships Dating Love Relationship Issues Hobbies and Crafts Crafts Drawing Games Education & Communication Communication Skills Personal Development Studying Personal Care and Style Fashion Hair Care Personal Hygiene Youth Personal Care School Stuff Dating All Categories Arts and Entertainment Finance and Business Home and Garden Relationship Quizzes Cars & Other Vehicles Food and Entertaining Personal Care and Style Sports and Fitness Computers and Electronics Health Pets and Animals Travel Education & Communication Hobbies and Crafts Philosophy and Religion Work World Family Life Holidays and Traditions Relationships Youth
- Browse Articles
- Learn Something New
- Quizzes Hot
- This Or That Game New
- Train Your Brain
- Explore More
- Support wikiHow
- About wikiHow
- Log in / Sign up
- Education and Communications
- Personal Development
- School Leadership
How to Write a Student Council Speech
Last Updated: January 17, 2024 Approved
This article was co-authored by Patrick Muñoz . Patrick is an internationally recognized Voice & Speech Coach, focusing on public speaking, vocal power, accent and dialects, accent reduction, voiceover, acting and speech therapy. He has worked with clients such as Penelope Cruz, Eva Longoria, and Roselyn Sanchez. He was voted LA's Favorite Voice and Dialect Coach by BACKSTAGE, is the voice and speech coach for Disney and Turner Classic Movies, and is a member of Voice and Speech Trainers Association. wikiHow marks an article as reader-approved once it receives enough positive feedback. This article has 125 testimonials from our readers, earning it our reader-approved status. This article has been viewed 2,014,801 times.
Being a member of the student council can help you help your school. However, it takes hard work to get into the student council . You need to craft a good speech that gives your classmates incentives to vote for you.
Sample Speeches

Writing the Introduction

- Do not merely start by saying, "My name is ___ and I'm running for student council." Your classmates will already know as much and this is not really a unique statement. There will be time to state the basic information after you've got the class's attention. [1] X Research source
- You can open with a question. Something like, "If there was one thing you could change about this school, what would it be?" Or a question that adds some humor , like, "I know what you're thinking. Why should I listen to this person?" and then proceed to lay out your credentials. Quotes on leadership, power, and guidance would also make good openings. However, make sure to double-check your sources and especially if you're finding quotes online. Many online quote databases, like Quote Garden or Brainy Quote, sometimes attribute quotes to the wrong sources. [2] X Research source
- If you're stuck, look up and read famous speeches. You can find many speeches from presidents, world leaders, civil rights activists, and others online. Pay attention to how they opened their speeches and ask yourself, "Was this interesting? Do I want to keep reading/listening? Why?" [3] X Research source

- State your name and grade in school. This may feel somewhat unnecessary if you go to a small school, but it's considered a formality. If you're missing this part of the speech, you may end up looking sloppy in comparison to other students. [5] X Research source
- State what you want. That is, what you're running for. Do you want to be the president , vice president , treasurer, secretary? Even if you think most students are aware of what position you're running for, make sure you state it here to remind them. [6] X Research source
- Try to keep this section brief as it's not as important as your qualifications and plans to improve the school . Even one sentence would suffice. For example, "My name is Ramona Hart, I'm in the 11th grade, and I'm running for treasurer of the student council."

- Any accomplishments relevant to the position warrant mentioning here. If you're running for secretary, for example, talk about your summer job filing papers in your uncle's law firm. If you're running for student council president, talk about your leadership experience being captain of the swim team. [7] X Research source
- While this section is important, try to keep it minimal. A couple of sentences laying out your qualifications is enough as the body of your speech is where you should spend the most time. For example, let's go back to the above example. From there, we could say, "I am currently enrolled in advanced placement algebra and I have been an honor roll student for three years. This knowledge of numbers and diligence qualifies me to have responsibility for finances for our student council." [8] X Research source
Writing the Body of the Speech

- You should list your ideas and then expand on them later in the body. It might take a bit of research to figure out what you want to change. Ask around the school, talking to students and teachers, and see where there's room for improvement. What are the concerns of the students? What are people happy with regarding the school? What would they like to see change? Asking these questions can help you get a sense of your audience and community.
- Remember, you should not make promises you cannot keep. Do not say anything just to get elected. While many students might want gum-chewing policies eliminated or for the lunch period to run twice as long, this is probably not necessary or possible. Try to focus on areas that seem important to keep your school running safely and efficiently. Concerns about things like bullying , academic standards, and extracurricular activities should be your concern over fun and games. [10] X Research source
- A good opening statement for your body would state the causes important to you and what you plan to do about them. For example, if you were running for president, you could say something like, "I understand we need to improve how we handle bullying, increase interest in extracurricular activities, and expand access to AP courses throughout the school. As your president, I would work to bring in speakers to talk about sensitivity in the classroom, increase advertising for basketball games and quiz bowl tournaments, and start a tutoring program to help students struggling with certain subjects." [11] X Research source

- Using the school library or computers, figure out the best means to tackle certain problems many schools face. How have other schools dealt with bullying? Poor test scores? Low interest in extracurricular activities? What can you reasonably do as a student council member to address these problems? [12] X Research source
- You do not have to have a point-by-point plan laid out, but a few sentences on some preliminary ideas can help you stand out from your peers. People are more inclined to vote for someone who's thought about how to solve problems in addition to identifying problems. [13] X Research source

Ending with a Strong Conclusion

- Do summarize, briefly, your qualifications but do not put the main focus on them. This is where you should sincerely state your passion. Students should not just vote for you because you'd do a good job but because you genuinely care about the school. State your passion for your community and how much you want to see other students succeed. Lots of students have high qualifications. You can set yourself apart by being a candidate who really cares. [16] X Research source

- Research what other student council speeches are like on video websites. This could help give you ideas.
Expert Q&A

- Only promise to do things that you really can do. Thanks Helpful 13 Not Helpful 2
- Practice reading your speech a few times, as you'll likely be nervous before giving it. Thanks Helpful 13 Not Helpful 2

- Even if you write a great speech, understand you may lose. Be prepared to lose graciously and sincerely congratulate the winning candidate. Thanks Helpful 101 Not Helpful 15
- Unlike in a governmental election, student council candidates should not attack each other, previous leaders, or other students. Otherwise, you could get into trouble and leave a bad impression on voters. Thanks Helpful 76 Not Helpful 16
You Might Also Like

- ↑ http://www.studentcouncilpro.com/student-council-speeches.html
- ↑ http://www.write-out-loud.com/student-council-speeches.html
- ↑ Patrick Muñoz. Voice & Speech Coach. Expert Interview. 12 November 2019.
About This Article

To write a student council speech, start with an attention-grabbing statement such as a question or a powerful quote about leadership. Next, briefly explain who you are, what position you are running for, and why you are running. Then list any relevant qualifications, such as a summer job. In the body of the speech, discuss at least 3 ways to improve the school. For this section, make sure not to make any promises you can’t keep. Finally, end by briefly reiterating your main points and asking for the students’ vote. To learn more about how to support your ideas and research for your speech, keep reading! Did this summary help you? Yes No
- Send fan mail to authors
Reader Success Stories

Did this article help you?

Sep 27, 2017

Kyla Harvie
Jun 11, 2017

Nov 6, 2021

Gavin Sedey
Oct 13, 2017

Featured Articles

Trending Articles

Watch Articles

- Terms of Use
- Privacy Policy
- Do Not Sell or Share My Info
- Not Selling Info
Get the free weekly newsletter
wikiHow's Best Advice on Dating & Love
- Speech Topics For Kids
- How To Write A Speech
How to Write a Speech: A Guide to Enhance Your Writing Skills
Speech is a medium to convey a message to the world. It is a way of expressing your views on a topic or a way to showcase your strong opposition to a particular idea. To deliver an effective speech, you need a strong and commanding voice, but more important than that is what you say. Spending time in preparing a speech is as vital as presenting it well to your audience.
Read the article to learn what all you need to include in a speech and how to structure it.
Table of Contents
- Self-Introduction
The Opening Statement
Structuring the speech, choice of words, authenticity, writing in 1st person, tips to write a speech, frequently asked questions on speech, how to write a speech.
Writing a speech on any particular topic requires a lot of research. It also has to be structured well in order to properly get the message across to the target audience. If you have ever listened to famous orators, you would have noticed the kind of details they include when speaking about a particular topic, how they present it and how their speeches motivate and instill courage in people to work towards an individual or shared goal. Learning how to write such effective speeches can be done with a little guidance. So, here are a few points you can keep in mind when writing a speech on your own. Go through each of them carefully and follow them meticulously.
Self Introduction
When you are writing or delivering a speech, the very first thing you need to do is introduce yourself. When you are delivering a speech for a particular occasion, there might be a master of ceremony who might introduce you and invite you to share your thoughts. Whatever be the case, always remember to say one or two sentences about who you are and what you intend to do.
Introductions can change according to the nature of your target audience. It can be either formal or informal based on the audience you are addressing. Here are a few examples.
Addressing Friends/Classmates/Peers
- Hello everyone! I am ________. I am here to share my views on _________.
- Good morning friends. I, _________, am here to talk to you about _________.
Addressing Teachers/Higher Authorities
- Good morning/afternoon/evening. Before I start, I would like to thank _______ for giving me an opportunity to share my thoughts about ________ here today.
- A good day to all. I, __________, on behalf of _________, am standing here today to voice out my thoughts on _________.
It is said that the first seven seconds is all that a human brain requires to decide whether or not to focus on something. So, it is evident that a catchy opening statement is the factor that will impact your audience. Writing a speech does require a lot of research, and structuring it in an interesting, informative and coherent manner is something that should be done with utmost care.
When given a topic to speak on, the first thing you can do is brainstorm ideas and pen down all that comes to your mind. This will help you understand what aspect of the topic you want to focus on. With that in mind, you can start drafting your speech.
An opening statement can be anything that is relevant to the topic. Use words smartly to create an impression and grab the attention of your audience. A few ideas on framing opening statements are given below. Take a look.
- Asking an Engaging Question
Starting your speech by asking the audience a question can get their attention. It creates an interest and curiosity in the audience and makes them think about the question. This way, you would have already got their minds ready to listen and think.
- Fact or a Surprising Statement
Surprising the audience with an interesting fact or a statement can draw the attention of the audience. It can even be a joke; just make sure it is relevant. A good laugh would wake up their minds and they would want to listen to what you are going to say next.
- Adding a Quote
After you have found your topic to work on, look for a quote that best suits your topic. The quote can be one said by some famous personality or even from stories, movies or series. As long as it suits your topic and is appropriate to the target audience, use them confidently. Again, finding a quote that is well-known or has scope for deep thought will be your success factor.
To structure your speech easily, it is advisable to break it into three parts or three sections – an introduction, body and conclusion.
- Introduction: Introduce the topic and your views on the topic briefly.
- Body: Give a detailed explanation of your topic. Your focus should be to inform and educate your audience on the said topic.
- Conclusion: Voice out your thoughts/suggestions. Your intention here should be to make them think/act.
While delivering or writing a speech, it is essential to keep an eye on the language you are using. Choose the right kind of words. The person has the liberty to express their views in support or against the topic; just be sure to provide enough evidence to prove the discussed points. See to it that you use short and precise sentences. Your choice of words and what you emphasise on will decide the effect of the speech on the audience.
When writing a speech, make sure to,
- Avoid long, confusing sentences.
- Check the spelling, sentence structure and grammar.
- Not use contradictory words or statements that might cause any sort of issues.
Anything authentic will appeal to the audience, so including anecdotes, personal experiences and thoughts will help you build a good rapport with your audience. The only thing you need to take care is to not let yourself be carried away in the moment. Speak only what is necessary.
Using the 1st person point of view in a speech is believed to be more effective than a third person point of view. Just be careful not to make it too subjective and sway away from the topic.
- Understand the purpose of your speech: Before writing the speech, you must understand the topic and the purpose behind it. Reason out and evaluate if the speech has to be inspiring, entertaining or purely informative.
- Identify your audience: When writing or delivering a speech, your audience play the major role. Unless you know who your target audience is, you will not be able to draft a good and appropriate speech.
- Decide the length of the speech: Whatever be the topic, make sure you keep it short and to the point. Making a speech longer than it needs to be will only make it monotonous and boring.
- Revising and practicing the speech: After writing, it is essential to revise and recheck as there might be minor errors which you might have missed. Edit and revise until you are sure you have it right. Practise as much as required so you do not stammer in front of your audience.
- Mention your takeaways at the end of the speech: Takeaways are the points which have been majorly emphasised on and can bring a change. Be sure to always have a thought or idea that your audience can reflect upon at the end of your speech.
How to write a speech?
Writing a speech is basically about collecting, summarising and structuring your points on a given topic. Do a proper research, prepare multiple drafts, edit and revise until you are sure of the content.
Why is it important to introduce ourselves?
It is essential to introduce yourself while writing a speech, so that your audience or the readers know who the speaker is and understand where you come from. This will, in turn, help them connect with you and your thoughts.
Leave a Comment Cancel reply
Your Mobile number and Email id will not be published. Required fields are marked *
Request OTP on Voice Call
Post My Comment

- Share Share
Register with BYJU'S & Download Free PDFs
Register with byju's & watch live videos.

How to Write a Winning Debate Speech

What is a Debate?
A classroom debate involves students delivering persuasive speeches to present and support their opinions on a given subject. This activity helps develop critical thinking and communication skills, enabling students to gain a more comprehensive grasp of various topics.
Debate speeches are written according to a set of rules so a moderator can assess their effectiveness and allow others to question or challenge their statements within a formal debate.
A classroom debate is not an unruly fight or pointless argument but a structured formal conversation on a chosen topic in which two teams argue for or against it to convince the neutral moderator that they hold the stronger position.
Debating is a form of persuasive communication, and while we will be sticking to the fundamentals of how to write a debating speech, we also have a great guide to persuasive essay writing that elaborates on specific persuasive techniques.
Complete Teaching Unit on Class Debating

This unit will guide your students to write excellent DEBATE SPEECHES and craft well-researched, constructed ARGU MENTS ready for critique from their classmates.
Furthermore, this EDITABLE UNIT will provide the TOOLS and STRATEGIES for running highly engaging CLASSROOM DEBATES.
How To Run A Classroom Debate
Before jumping in headfirst to write your debating speech, ensure you understand how a debate is run to maximise your strategy and impact when it counts.
Debates occur in many different contexts, such as public meetings, election campaigns, legislative assemblies, and as entertainment on television shows. These contexts determine the specific structure the debate will follow.
This guide provides a basic step-by-step debate structure we can comfortably run with students in a classroom. By familiarizing students with this structure, they will effortlessly transition to other debate frameworks.
Running a classroom debate can be an engaging and educational activity that helps students develop critical thinking, communication, and research skills. Here’s a step-by-step guide on how to organize and facilitate a successful classroom debate:
1. Choose a Topic For Your Debate.
Also called a resolution or a motion , the topic is sometimes chosen to debate. This is usually the case in a school activity to practice debating skills.
The resolution or motion is usually centered around a true or false statement or a proposal to change the current situation. Often, the motion starts, ”This House believes that….”
Select a topic relevant to your curriculum and the students’ interests. Ensure that it is debatable and has multiple perspectives. Further down this article, you can find a list of popular classroom debating topics.
2. Form Two Debating Teams
Two teams of three speakers each are formed. These are referred to as ‘ The House for the Motion ’ or the ‘ Affirmative ’ team and ‘The House Against the Motion ’ or the ‘ Negative ’ team.
Preparation is an essential aspect of debating. The speech and debate team members will need time to research their arguments, collaborate, and organize themselves and their respective roles in the upcoming debate.
They’ll also need time to write and rehearse their speeches. The better prepared and coordinated they are as a team, the greater their chances of success in the debate.
3. Assign Roles to Students.
Each team member should have a specific role, such as speaker, researcher , or rebuttal specialist . This encourages teamwork and ensures that each student is actively involved.
4. Research and Preparation:
- Allocate time for teams to research and prepare their arguments. Encourage students to use multiple sources, including books, articles, and reputable websites. Make sure you read our complete guide to powerful student research strategies.
5. Set Debate Format:
- Define the debate format, including the structure of each round. Common formats include opening statements, cross-examination, rebuttals, and closing statements.
6. Establish Rules:
- Set ground rules for the debate, such as time limits for each speaker, etiquette, guidelines for respectful communication, and consequences for rule violations.
7. Conduct a Practice Debate:
- Before the actual debate, conduct a practice round. This helps students become familiar with the format and allows you to provide feedback on their arguments and presentation skills.
- On the day of the debate, set up the classroom to accommodate the format. Ensure that each round has a clear structure, and designate a timekeeper to keep the debate on schedule.
9. Facilitate Q&A Sessions:
- After each team presents their arguments, allow time for questions and cross-examination. This encourages critical thinking and engagement among the students.
10. Evaluate and Debrief:
- After the debate, provide constructive feedback to each team. Discuss the strengths and weaknesses of their arguments, presentation skills, and teamwork. Also, please encourage students to reflect on what they learned from the experience.
- Have a class discussion about the debate, exploring different perspectives and opinions. This can deepen students’ understanding of the topic and enhance their critical thinking skills.
Consider integrating the debate topic into future lessons or assignments. This reinforces the learning experience and allows students to delve deeper into the subject matter.
Remember to create a supportive and respectful environment throughout the debate, emphasizing the importance of listening to opposing views and engaging in constructive dialogue.
Each speaker takes a turn making their speech, alternating between the House for the Motion, who goes first, and the House Against the Motion. Each speaker speaks for a pre-agreed amount of time.
Ensure your debate is held in front of an audience (in this case, the class), and occasionally, the audience is given time to ask questions after all the speeches have been made.
Finally, the debate is judged either by moderators or by an audience vote.
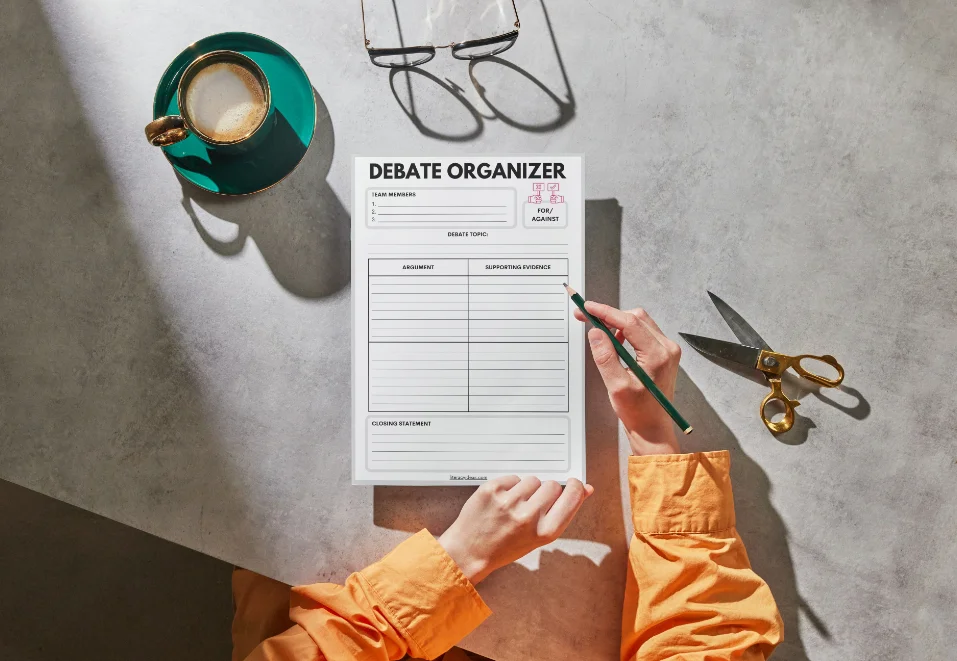
Download our Debate Organizer
Stay fousssed with this handy template to keep all your ideas organized.
How To Write A Debate
How to start a debate speech.
In highly competitive speech and debate tournaments, students are only provided the topic on the day, and limited time is allowed for preparation, but this is not recommended for beginners.
Regardless of the stakes of your classroom debate, the speechwriting process always begins with research. Thorough research will provide students with both the arguments and the supporting evidence for their position on a topic and generate forward-thinking about what their opponents might use against them.
Writing Your Introduction
The purpose of the introduction in a debate speech is to achieve several things:
- Grab the attention of the audience,
- Introduce the topic
- Provide a thesis statement
- Preview some of the main arguments.
Grab The Attention Of Your Audience With Strong Hooks
Securing the audience’s attention is crucial, and failure to do this will have a strong, negative impact on how the team’s efforts will be scored as a whole. Let’s explore three proven strategies to hook your audience and align their thinking to yours.
Introduce Your Topic With Efficiency and Effectiveness
Once the audience’s attention has been firmly grasped, it’s time to introduce the topic or the motion. This should be done straightforwardly and transparently to ensure the audience understands the topic of the debate and the position you are approaching it from.
For example, if the topic of the debate was school uniforms, the topic may be introduced with:
Provide Your Thesis Statement
A thesis statement is a concise declaration summarizing the points and arguments of your debating speech.
- It presents a clear stance on a topic and guides the reader on what to expect in the content.
- A good thesis statement is debatable and allows for opposing viewpoints and discussion.
- It serves as a roadmap for the writer, ensuring coherence and focus in the piece.
- It helps the audience understand the purpose and direction of the work from the beginning.
The thesis statement should express the student’s or the team’s position on the motion. Clearly explaining the speaker’s side of the debate. An example can be seen here.
Provide A Preview Of Your Arguments
The final part of the introduction section of a debate speech involves previewing the main points of the speech for the audience.
There is no need to go into detail with each argument here; that’s what the body of the speech is for. It is enough to provide a general thesis statement for each argument or ‘claims’ – (more on this to follow).
Previewing the arguments in a speech is especially important as the audience and judges only get one listen to a speech – unlike a text, which can be reread as frequently as the reader likes.
debate introduction examples for students
Attention grabbers task.
After explaining the different types of attention grabbers and the format for the rest of the introduction to your students, challenge them to write an example of each type of opening for a specific debate topic.
When they’ve finished writing these speech openings, discuss with the students which one best fits their chosen topic. Then, they can continue by completing the rest of the introduction for their speech using the format described above.
You might like to try a simple topic like “Homework should be banned.” you can choose from our collection further in this article.
Writing T he Body of the Speech
The body paragraphs are the real meat of the speech. They contain the in-depth arguments that make up the substance of the debate, and How well these arguments are made will determine how the judges will assess each speaker’s performance, so it’s essential to get the structure of these arguments just right.
Let’s take a look at how to do that.
How to structure an Argument
With the introduction out of the way, it’s time for the student to get down to the nitty-gritty of the debate – that is, making compelling arguments to support their case.
There are three main aspects to an argument in a debate speech. They are:
- The Warrant
Following this structure carefully enables our students to build coherent and robust arguments. Ttake a look at these elements in action in the example below.
Brainstorming Arguments
Present your students with a topic and, as a class, brainstorm some arguments for and against the motion.
Then, ask students to choose one argument and, using the Claim-Warrant-Impact format, take a few moments to write down a well-structured argument that’s up to debate standard.
Students can then present their arguments to the class.
Or, you could also divide the class along pro/con lines and host a mini-debate!
Concluding a Debate Speech
The conclusion of a speech or a debate is the final chance for the speaker to convey their message to the audience. In a formal debate that has a set time limit, the conclusion is crucial as it demonstrates the speaker’s ability to cover all their material within the given time frame.
Avoid introducing new information and focus on reinforcing the strength of your position for a compelling and memorable conclusion.
A good conclusion should refer back to the introduction and restate the main position of the speaker, followed by a summary of the key arguments presented. Finally, the speaker should end the speech with a powerful image that will leave a lasting impression on the audience and judges.

Examples of strong debate Conclusions
The Burden of the Rejoinder
In formal debates, the burden of the rejoinder means that any time an opponent makes a point for their side, it’s incumbent upon the student/team to address that point directly.
Failing to do so will automatically be seen as accepting the truth of the point made by the opponent.
For example, if the opposing side argues that all grass is pink, despite how ridiculous that statement is, failing to refute that point directly means that, for the debate, all grass is pink.
Our students must understand the burden of the rejoinder and ensure that any points the opposing team makes are fully addressed during the debate.
The Devils Advocate
When preparing to write their speech, students should spend a significant proportion of their team collaborating as a team.
One good way to practice the burden of the rejoinder concept is to use the concept of Devil’s Advocate, whereby one team member acts as a member of the opposing team, posing arguments from the other side for the speaker to counter, sharpening up their refutation skills in the process.
20 Great Debating Topics for Students
- Should cell phones be allowed in schools?
- Is climate change primarily caused by human activities?
- Should the voting age be lowered to 16?
- Is social media more harmful than beneficial to society?
- Should genetically modified organisms (GMOs) be embraced or rejected?
- Is the death penalty an effective crime deterrent?
- Should schools implement mandatory drug testing for students?
- Is animal testing necessary for scientific and medical advancements?
- Should school uniforms be mandatory?
- Is censorship justified in certain circumstances?
- Should the use of performance-enhancing drugs be allowed in sports?
- Is homeschooling more beneficial than traditional schooling?
- Should the use of plastic bags be banned?
- Is nuclear energy a viable solution to the world’s energy needs?
- Should the government regulate the fast food industry?
- Is social inequality a result of systemic factors or individual choices?
- Should the consumption of meat be reduced for environmental reasons?
- Is online learning more effective than traditional classroom learning?
- Should the use of drones in warfare be banned?
- Is the legalization of marijuana beneficial for society?
These topics cover a range of subjects and offer students the opportunity to engage in thought-provoking debates on relevant and impactful issues.
OTHER GREAT ARTICLES RELATED TO DEBATING
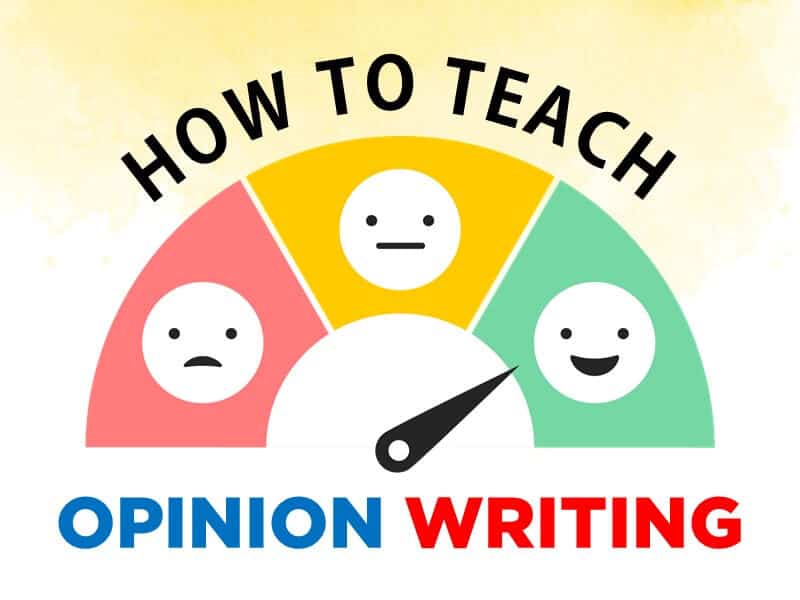
The Ultimate Guide to Opinion Writing for Students and Teachers
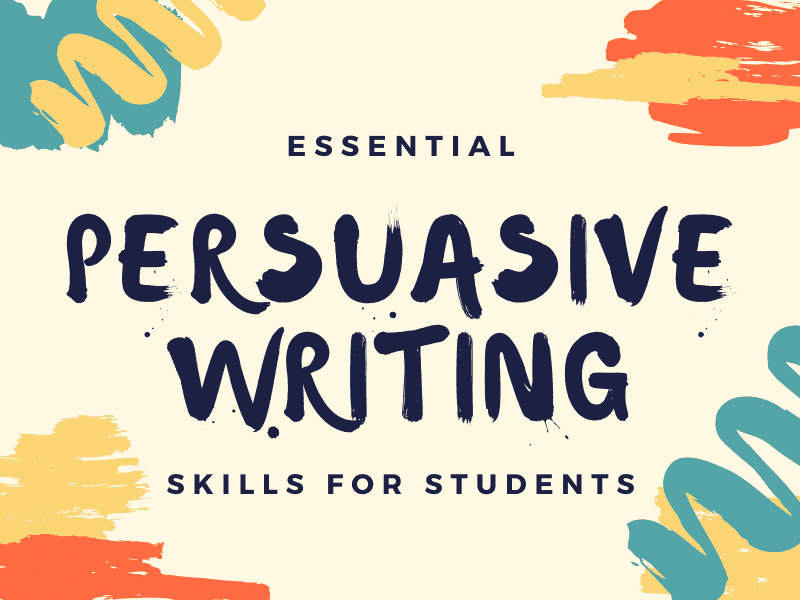
Top 5 Persuasive Writing Techniques for Students
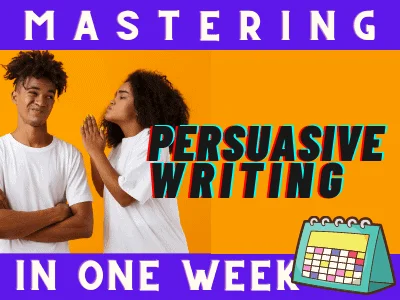
5 Top Persuasive Writing Lesson Plans for Students and Teachers
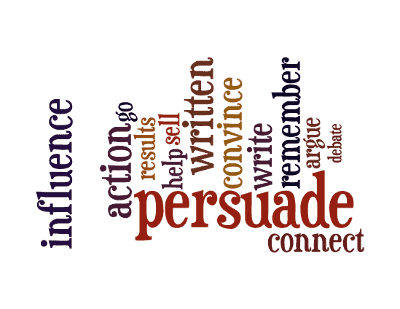
23 Persuasive writing Topics for High School students
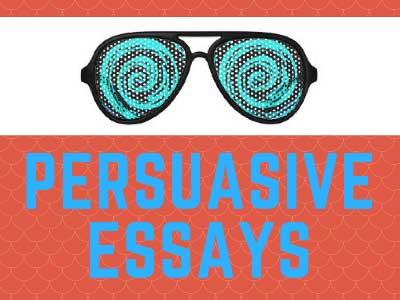
How to Write Perfect Persuasive Essays in 5 Simple Steps
Debating strategies for students.
Research and preparation are essential to ensure good performance in a debate. Students should spend as much time as possible drafting and redrafting their speeches to maximize their chances of winning. However, a debate is a dynamic activity, and victory cannot be assured by pre-writing alone.
Students must understand that the key to securing victory lies in also being able to think, write (often in the form of notes), and respond instantly amid the turmoil of the verbal battle. To do this, students must understand the following keys to victory.
When we think of winning a debate, we often think of blinding the enemy with the brilliance of our verbal eloquence. We think of impressing the audience and the judges alike with our outstanding oratory.
What we don’t often picture when we imagine what a debate winner looks like is a quiet figure sitting and listening intently. But being a good listener is one of our students’ most critical debating skills.
If students don’t listen to the other side, whether by researching opposing arguments or during the thrust of the actual debate, they won’t know the arguments the other side is making. Without this knowledge, they cannot effectively refute the opposition’s claims.
Read the Audience
In terms of the writing that happens before the debate takes place, this means knowing your audience.
Students should learn that how they present their arguments may change according to the demographics of the audience and/or judges to whom they will be making their speech.
An audience of retired school teachers and an audience of teen students may have very different responses to the same arguments.
This applies during the actual debate itself too. If the student making their speech reads resistance in the faces of the listeners, they should be prepared to adapt their approach accordingly in mid-speech.
Practice, Practice, Practice
The student must practice their speech before the debate. There’s no need to learn it entirely by heart. There isn’t usually an expectation to memorize a speech entirely, and doing so can lead to the speaker losing some of their spontaneity and power in their delivery. At the same time, students shouldn’t spend the whole speech bent over a sheet of paper reading word by word.
Ideally, students should familiarize themselves with the content and be prepared to deliver their speech using flashcards as prompts when necessary.
Another important element for students to focus on when practising their speech is making their body language, facial expressions, and hand gestures coherent with the verbal content of their speech. One excellent way to achieve this is for the student to practice delivering their speech in a mirror.
And Finally…
Debating is a lot of fun to teach and partake in, but it also offers students a valuable opportunity to pick up some powerful life skills.
It helps students develop a knack for distinguishing fact from opinion and an ability to assess whether a source is credible or not. It also helps to encourage them to think about the other side of the argument.
Debating helps our students understand others, even when disagreeing with them. An important skill in these challenging times, without a doubt.
Debating Teaching Strategies
Clearly Define Debate Roles and Structure when running speech and debate events: Clearly define the roles of speakers, timekeepers, moderators, and audience members. Establish a structured format with specific time limits for speeches, rebuttals, and audience participation. This ensures a well-organized and engaging debate.
- Provide Topic Selection and Preparation Time: Offer students a range of debate topics, allowing them to select a subject they are passionate about. Allocate ample time for research and preparation, encouraging students to gather evidence, develop strong arguments, and anticipate counterarguments.
- Incorporate Scaffolded Debating Skills Practice: Before the actual debate, engage students in scaffolded activities that build their debating skills. This can include small group discussions, mock debates, or persuasive writing exercises. Provide feedback and guidance to help students refine their arguments and delivery.
- Encourage Active Listening and Note-taking during speech and debate competitions: Emphasize the importance of active listening during the debate. Encourage students to take notes on key points, supporting evidence, and persuasive techniques used by speakers. This cultivates critical thinking skills and prepares them for thoughtful responses during rebuttals.
- Facilitate Post-Debate Reflection and Discussion: After the debate, facilitate a reflection session where students can share their thoughts, lessons learned, and insights gained. Encourage them to analyze the strengths and weaknesses of their arguments and engage in constructive dialogue. This promotes metacognitive skills and encourages continuous improvement.
By following these tips, teachers can create a vibrant and educational debate experience for their students. Through structured preparation, active engagement, and reflective discussions, students develop valuable literacy and critical thinking skills that extend beyond the boundaries of the debate itself.
A COMPLETE UNIT FOR TEACHING OPINION WRITING
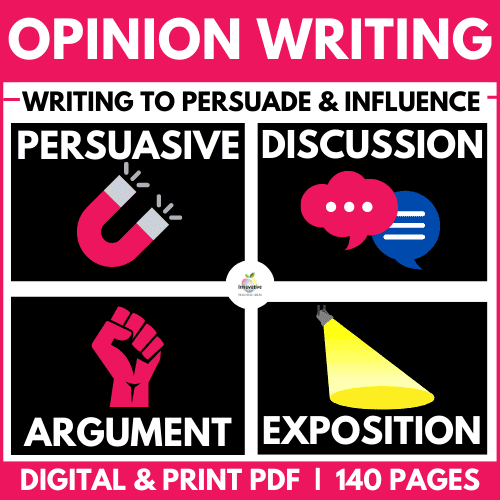
Teach your students to write EXCELLENT PERSUASIVE ESSAYS and master INFLUENTIAL WRITING SKILLS using PROVEN TEACHING STRATEGIES with this 140-PAGE UNIT.
ALL RESOURCES AND ASSESSMENT TOOLS INCLUDED – NO PREP REQUIRED.
30+ 5-star Ratings ⭐⭐⭐⭐⭐
- Write my thesis
- Thesis writers
- Buy thesis papers
- Bachelor thesis
- Master's thesis
- Thesis editing services
- Thesis proofreading services
- Buy a thesis online
- Write my dissertation
- Dissertation proposal help
- Pay for dissertation
- Custom dissertation
- Dissertation help online
- Buy dissertation online
- Cheap dissertation
- Dissertation editing services
- Write my research paper
- Buy research paper online
- Pay for research paper
- Research paper help
- Order research paper
- Custom research paper
- Cheap research paper
- Research papers for sale
- Thesis subjects
- How It Works
100 Best How To Speech Topics & Ideas In 2023
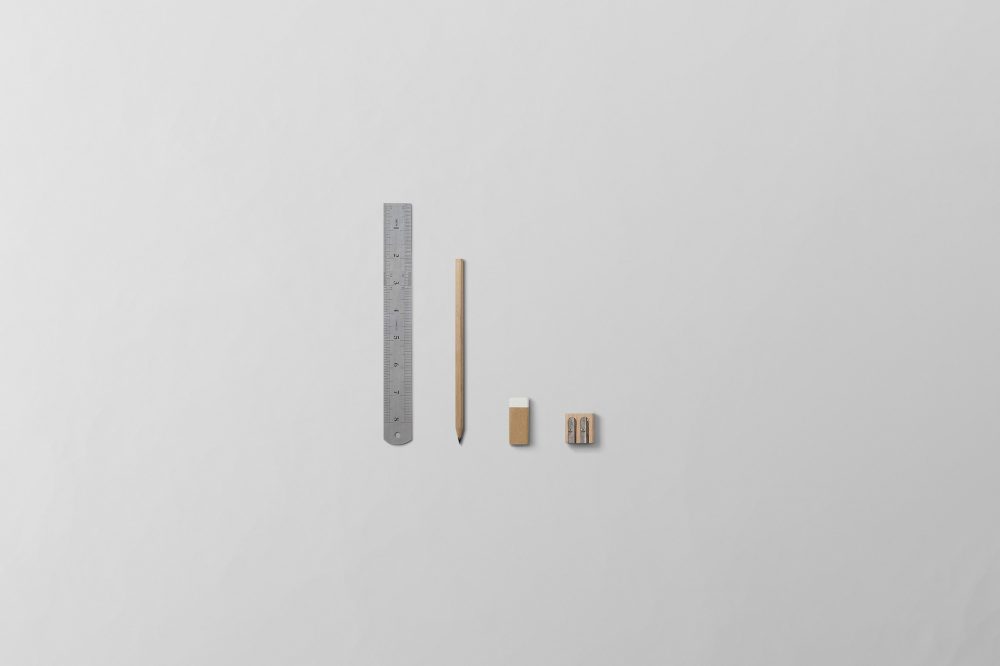
How to topics make students feel like experts. They also allow them to practice non-fiction writing. That’s because these topics require them to explain how a person can do something. It can be something simple like how to follow more topics on Pinterest or something complex like how to create a nuclear reactor.
When writing about how to essay topics, students should dissect processes from the beginning to the end. They should also include all the necessary information to enable the reader to achieve their desired results. Essentially, essays and speeches on these topics should guide readers into doing something successfully.
The Purpose of How To Speech Topics
Good how-to topics make learners more confident and competent nonfiction writers. Some students consider this as their writing genre. These topics describe how certain tasks are completed. Something good about this writing genre is that it allows learners to describe processes that they are familiar with.
This enables learners to focus on writing while enjoying the experience that’s enjoyed by experts. They also feel good about communicating something meaningful to their audiences. In most cases, students are asked to write how-to speeches. That means they have to choose how to topics for demonstration speech. And, this genre has many topics that students can choose from. Here are some of the major categories of how to speech ideas.
How To Speeches Topics for High School Students
Some high school teachers ask students to write speeches demonstrating how certain things are done. If you’ve been asked to write on such a topic, here are good how-to speech topics to choose from.
- How to write an admission letter for university or college
- How to remain safe in an unfamiliar or new environment
- How to make new friends at a new college
- How to choose a college or university
- How to choose a study program
- How to choose a career
- How to choose the best game
- How to become a public speaker
- How to choose activities that improve self-esteem and confidence
- How plants produce and use oxygen
- How to put off something you don’t like without hurting others
- How to prepare for a marathon or a long race
- How to get a scholarship to study overseas
- How to stay fit in high school
- How to grow flowers indoors or in a garden
- How to create something like a painting or building at home
- How to prepare French fries at home
- How to prepare for college
- How to search for academic articles on the internet
- How to prepare for a trip with family
These are great how-to speech topics that can earn top grades for high school students when researched and written properly. It’s, however, important that learners choose the topics they are familiar with and comfortable writing about.
How To Topics for Second Grade
Second grade is the time when children are developing writing skills. At this time, children start to express their opinions and recount narratives. They can also provide instructions in writing. Here are some of the best topics of how to teach writing to second-grade children. Asking second-grade children to write about these topics will engage them in writing and spark their creativity.
- How to be kind to other people
- How to make your birthday special and memorable
- How to build a beautiful house
- How to read a bedtime story
- How to tell a story
- How to make chocolate
- How to play your favorite games
- How to tie your shoes
- How to keep a friend
- How to take care of your pet
- How to get a good school report card
- How to make a friend
- How to prepare for dinner
- How to sharpen a pencil
- How to blow bubbles
- How to draw a picture
- How to paint the best picture
- How to tidy your room
- How to tidy your desk
- How to give a pet a bath
These are great how to presentation topics that can enable second-grade children to express their opinions with simple words and improve their writing skills.
How To Speeches Topics for College Students
College time provides a chance to sharpen writing skills. This is the place where students learn to research, analyze, organize, and present solid ideas. Here, students are expected to write on how to process speech topics that can be used to do something professionally.
Here are great how to demonstration speech topics community college students can choose from:
- How to use a Smartphone to complete homework
- How to avoid peer pressure
- How to avoid recurring nightmares
- How to write a great essay
- How to write a research paper
- How to look stylish and fashionable in college
- How to choose a major
- How to avoid procrastination in college
- How to avoid stressful situations in college
- How to finish Aleks topics fast
- How to study for exams
- How to write a great film review
- How to start a business after college
- How to improve your writing skills
- How to improve your public speaking skills
- How to enhance your leadership skills
- How to survive a recession
- How to start an online business
- How to avoid drug addiction in college
- How to learn a second language in college
These are also amazing how to topics for speech or presentation. However, they should be researched properly to come up with informative pieces.
Topics for How To Speech for University
Maybe you’ve been asked to write a speech and present it to demonstrate how to do something. Here are amazing how to process speech topics that you can choose from.
- How to survive a pandemic
- How to introduce a topic for an essay
- How to write a narrative essay
- How to choose an essay topic
- How to devise a lesson plan for teaching preschool children
- How to campaign for a leadership position
- How to search for academic journals on the internet
- How to write a book review
- How to research a topic before writing a paper
- How to effectively manage finances and save money
- How to perform a qualitative study using people as your subjects
- How to avoid stress in the workplace
- How to start and run an online cosmetic business
- How to stay fit during a pandemic
- How to prepare for a new job
- How to prepare for an interview
- How to install new software on a laptop or desktop computer
- How to stay fit without skipping essential meals
Funny How To Topics
Perhaps, you want to write the most interesting speech or essay. In that case, consider the following topics.
- How to fail exams easily
- How to become the best in procrastination
- How to look more intelligent
- How to use hobbies to judge others
- How to catch a liar
- How to persuade your parents to do homework for you
- How to use the horoscope to explain failures
- How to boost your IQ by playing computer games
- How to convince your friends to pay your bills
- How to determine the intelligence level of your pet
- How to teach your pet to talk
- How to become the most annoying person in your school
- How to make your parents accommodate you when you are already 30 years old
- How to ensure that your parents do not spend a lot of time on social media
- How to make your first date a total failure
- How to be the happiest person in your family
- How to make your girlfriend or boyfriend love you forever
- How to make other people hate you
- How to make people love you
- How to push an annoying person away
Every student knows how it feels to walk into hot topics. If you don’t want to struggle to search for the information required to write an essay or a speech, consider topics in this category. Ask our thesis writers to help you, if you cannot make your choice.
Choosing topics in this genre can mark the beginning of the nonfiction writing journey for most students. Nevertheless, learners should know how to come up with story ideas and research them properly to come up with informative, solid essays or speeches.
Leave a Reply Cancel reply
My Speech Class
Public Speaking Tips & Speech Topics
“How To” Speech Topics, Ideas & Examples
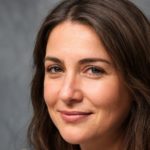
Amanda Green was born in a small town in the west of Scotland, where everyone knows everyone. I joined the Toastmasters 15 years ago, and I served in nearly every office in the club since then. I love helping others gain confidence and skills they can apply in every day life.
Want to speak in front of an audience but are terrified of freezing or being boring? I know the feeling. If you’ve ever had to give a “how-to” speech, you know that it can be incredibly intimidating. It also entails coming up with how-to speech ideas and creative examples.
After all, how will they finish listening if the audience isn’t hooked by what you’re saying right away? To help ease your worries (and inspire those ideas!), here’s everything you need on “how-to” speeches: topics, samples & potential pitfalls — so read ahead!
Writing a How-to Speech

The speaker needs to consider several important factors when preparing to give a how-to speech. The how-to speech should identify the problem that needs to be resolved, explain how the problem can be fixed with a step-by-step approach, and list any potential obstacles the audience may face.
It is also important for speakers to choose how-to speech topics that are of interest or relevance to their target audience. This will ensure maximum engagement and retention throughout the speech.
How-to Speech Ideas That Are Funny
When it comes to funny “how-to” speeches, the possibilities are endless! Whether talking about how to make a perfect paper airplane or how to be the life of the party, humor can enhance your presentation and help keep your audience engaged.
Try using puns, silly props, or even anecdotes that provide insight into the process while still making people laugh. The goal is to not only impart valuable knowledge but also entertain your listener.
With some creativity, you can develop a humorous speech that achieves a comedic effect and explains its topic.
Can We Write Your Speech?
Get your audience blown away with help from a professional speechwriter. Free proofreading and copy-editing included.
Interesting How-to Topics
When asked to present a speech, many people immediately search for the most interesting ideas for a how-to speech. From tidying up after the biggest mess to baking a delicious cake, speeches about how to do something can be informative and entertaining.
While it may seem challenging to come up with fresh ideas, an infinite number of topics could be used. Some great suggestions include teaching viewers how to create a simple budget plan , explaining plant-based diets and their benefits, explaining how to instruct others effectively, or even how to ace an interview.
No matter what topic you go with, remember that the important thing is to be passionate and provide audiences with detailed instructions. When done correctly, choosing an interesting “how-to” topic for your speech will surely have your audience applauding in no time!
Choosing a Demonstration Speech Topic
Choosing a demonstration speech topic may seem daunting, but it can be simple and enjoyable with the right guidance. Identifying the topics appropriate for a demonstration speech is the first step in navigating this process.
Demonstration topics should be grounded in facts and provide pertinent knowledge about your expertise or interest. They should also focus on something that can be demonstrated in a tangible way, such as making a craft or performing an experiment.
- Your Interests
Choosing engaging good how-to speech topics based on one’s interests can be daunting yet rewarding. Taking the time to reflect on potential topics and thinking carefully about what topics truly interest you is fundamental to assembling a captivating presentation.
As such, it is paramount to begin by reflecting upon your hobbies and leisure activity ideas that you find most intriguing, as well as any activities or tasks you feel passionate about sharing with others.
Additionally, curating ideas from other media sources, such as newspaper articles or magazines, helps provide further insight into new and novel subjects or angles that can motivate your research process.
Only through this deliberate effort to understand our interests and apply them creatively towards developing a worthwhile “how-to” speech topic can we find the contentment of presenting a presentation with which listeners will engage.
- Who the Speech Is For
Choosing a “how-to” speech topic is critical in delivering an effective presentation. When selecting your topic, it’s important to keep the audience in mind.
Decide who your speech is for and make sure it is relevant to their interests. Select something specific that can benefit them or be beneficial in a general sense. Choose something the audience wants to learn about and that you are comfortable presenting. This will ensure your presentation skills are on display, as well as your knowledge about the topic.
Ensure your topic has enough depth for you to use additional research as support. This will strengthen the content of your presentation and demonstrate personal effort.
Ultimately, with careful consideration of who your speech is addressed to, you can craft a “how-to” topic that adds value both for yourself and your audience.
- The Setting of the Speech
Choosing a “how-to” speech topic based on the setting of the speech is an important step in preparing for an effective presentation.
It is essential to consider the purpose of the speech, the audience’s interests and needs, and any limitations or restrictions that may be present to construct an appropriate topic and choose relevant information to include.
Anticipating questions or topics of interest related to the audience is helpful when selecting a relevant topic.
- The Time You’ve Got to Prepare the Speech
Deciding on a “how-to” speech topic can be daunting. But the task becomes much more manageable, considering how much time you have to prepare for the presentation. Start by narrowing down the possibilities based on how much time you have.
If you have more time to prepare, opt for something more challenging. If you have only a short amount of time, choose a simpler topic.
Once you have determined the complexity level and estimated preparation time, consider your interests to find a topic that is both engaging to yourself and hopefully your audience.
As with any speech or presentation, researching and practicing before the actual event will greatly benefit your delivery and engagement with your audience.
Although finalizing a speech topic for a “how-to” presentation can be stressful, understanding constraints and utilizing interests will help make this process easier.
- The Time You’ve Got to Give the Speech
When selecting a “how-to” speech topic, the amount of time you have to give the presentation should be considered. It is wise to carefully analyze the time frame constraints of the assignment and assess which topics can be thoroughly explored in the allocated duration.
Attempting to cover too much material within a limited timeframe may lead to rushed communication and limit your opportunity to expand on the subject matter. Instead, select a focus that allows space for further discussion but remains concise enough to explore properly within your allotted time.
It is also advantageous to choose a topic that naturally interests you. This will heighten enthusiasm and engagement throughout your presentation, thus helping create an impactful delivery. Doing so will ensure you stay on track and avoid exceeding the assigned timeframe.
- The Guidelines for Assessment
When choosing a “how-to” speech topic, it is important to ensure that the topic meets all of the guidelines for assessment. It is a good idea to consider current and relevant topics, informative, interesting, and new, demonstrating the speaker’s understanding of their subject matter.
Choosing a topic with an appropriate difficulty level can help ensure that your speech will be comprehensive and engaging for your audience.
Demonstrative Speech Topics

Selecting topics for speeches comes down to choosing something you both know and can present effectively. As you brainstorm possible show-and-tell ideas, narrow the topics until you arrive at one that is interesting to you and your audience and fulfills all criteria needed for an effective demonstration presentation.
Once you’ve chosen a successful topic, preparing the speech will become much simpler, allowing you to demonstrate confidently and successfully.
Here are some easy demonstrative speech ideas for how-to.
Animals/Pets
Giving a speech to educate an audience about how to care for animals/pets can be an enriching experience.
It is important to research and thoroughly understand the topic to provide the audience with accurate and applicable information. This will entail researching different animal species and their specific characteristics, needs, and behaviors.
Moreover, ample time must be allocated for preparation so all aspects of proper pet-keeping can be explained clearly and confidently.
Topics ideas could range from a persuasive speech on how to pick the best breed to an informative speech on pet health.
Gardens/Yards
Talking about creating and maintaining a garden or a yard requires proper preparation to ensure that the information relayed is accurate and beneficial. Researching various gardening elements beforehand will provide a strong base for discerning audiences to build on.
Be sure to present topics such as plant selection, design ideas, pest prevention, watering methods, and other environmental factors in an organized fashion that is easy for your listeners to follow.
Offering clear instructions throughout the speech with examples showing the steps in action can help ensure that your audience walks away feeling confident about their newly acquired knowledge.
Topics could cover how to identify poisonous plants, how to get rid of garden pests, how to make an indoor herb garden, and even how to make garden art.
To give an effective “how-to” speech about crafts, the speaker should start by introducing themselves and properly framing the topic. They should provide a brief overview of what the audience will learn in their presentation and then begin with the most basic information, slowly building up to more complex concepts.
Crafting often has visual aid speech topics. At every stage of presenting, visuals should be provided for illustration. Visuals could range from diagrams or step-by-step photographs showing how a craft is made to video or actual physical models.
Once all of this information has been presented, the speaker should summarize the key points covered and allow time for questions from the audience before concluding their presentation.
Games/Sports
Giving a “how-to” speech about games/sports is an excellent way to share knowledge and teach important concepts. It requires the speaker to do comprehensive research and prepare in advance.
To give an effective “how-to” speech, start by introducing the game/sport accurately and make sure to tailor the presentation for your specific audience. Additionally, focus on one topic at a time, explain each step with vivid examples, and use visuals like charts or diagrams if possible.
Once you have covered all steps necessary for playing the game/sport, end the speech concisely and provide resources that can be used after the presentation.
Topics here could range from how to perfect your golf swing to even how to teach basketball.
Food And More
Delivering a successful “how-to” speech about food and drinks requires careful preparation. First, it is important to do research on the topic so that you have an understanding of the background information.
Once this has been accomplished, the next step should be to focus on a specific field within the topic and craft your presentation around this selected focus. Be sure to collect illustrations, charts, or photographs relevant to your chosen material, as these visual elements can make all the difference in bringing your words to life.
How to Structure a How-to Speech
Structuring a “how-to” speech can seem intimidating, but it doesn’t have to be. Firstly, you should brainstorm the steps needed to accomplish your desired outcome with as much detail as possible. Secondly, organize the steps in chronological order.
This will give the rhythm of your speech a natural flow. Then, begin by introducing yourself and the topic by providing background information on why it is important.
Following completion of the step-by-step instructions, summarize what was just explained and explain why it matters in general terms. Finally, address any foreseeable issues and offer solutions to avoid them.
How-to Speech Template
A “how-to” speech template is an effective method to prepare a speech. This is especially useful when speaking on topics that may be largely unfamiliar to the audience, as it provides a straightforward structure for organizing the material.
The basic format of this type of speech involves:
- Introducing the topic.
- Describing the purpose and benefits of knowing more about it.
- Decomposing the subject matter into easily digestible sections.
- Concluding with a summary and reflection on what has been covered.
Additionally, including visual aids and personal anecdotes can further add interest and clarity to speeches, helping ensure everyone remains engaged throughout the presentation. Use this blank demonstration speech outline to craft your own any time!
To Sum It Up
Giving a great how-to speech is mostly about choosing the right topic. As how-to speeches often require visuals such as PowerPoint slides and handouts, these should be reviewed pre-speech to guarantee smooth delivery during the presentation itself. Speaking confidently and clearly while allowing time for questions and feedback is also essential in delivering an effective how-to speech.
Father of the Groom Speech – Best Tips, Ideas & Samples
9 Best Veterans Day Speech Ideas & Examples
Leave a Comment
I accept the Privacy Policy
Reach out to us for sponsorship opportunities
Vivamus integer non suscipit taciti mus etiam at primis tempor sagittis euismod libero facilisi.
© 2024 My Speech Class
Advertisement
Supported by
Biden Announces Student Debt Relief for Millions in Swing-State Pitch
During an appearance in Wisconsin, President Biden said 10 million borrowers could see debt relief of at least $5,000. The plan could help rally support among young voters.
- Share full article
Biden Announces New Plan for Student Debt Relief
President biden announced a large-scale effort to help pay off federal student loans for more than 20 million borrowers..
Today, I’m proud to announce five major actions to continue to relieve student debt for more than 30 million Americans since I started my administration. And starting this fall, we plan to deliver up to $20,000 in interest relief to over 20 million borrowers and full forgiveness for millions more. [applause] I will never stop to deliver student debt relief and hardworking Americans. And it’s only in the interest of America that we do it. And again, it’s for the good of our economy that’s growing stronger and stronger, and it is, by freeing millions of Americans from this crushing debt of student debt. It means they can finally get on with their lives instead of being put — their lives being put on hold.

By Michael D. Shear
Reporting from Madison, Wis.
President Biden on Monday announced a large-scale effort to help pay off federal student loans for tens of millions of American borrowers, seeking an election-year boost by returning to a 2020 campaign promise that was blocked by the Supreme Court last year.
Mr. Biden’s new plan would reduce the amount that 25 million borrowers still owe on their undergraduate and graduate loans. It would wipe away the entire amount for more than four million Americans. Altogether, White House officials said, 10 million borrowers would see debt relief of $5,000 or more.
“While a college degree still is a ticket to the middle class, that ticket is becoming much too expensive,” Mr. Biden said during a speech to a small but enthusiastic audience filled with supporters. “Today, too many Americans, especially young people, are saddled with too much debt.”
Mr. Biden announced the plan in Madison, Wis., the capital of a critical swing state and a college town that symbolizes the president’s promise to make higher-education affordability a cornerstone of his economic agenda.
But it is a promise he has so far failed to achieve, largely because of legal challenges from Republicans and other critics. They accuse Mr. Biden of unlawfully using his executive authority to enact a costly transfer of wealth from taxpayers who have not taken out federal student loans to those who have.
Officials did not say how much the new plan would cost in coming years, but critics have said it could increase inflation and add to the federal debt by billions of dollars.
Mr. Biden said his new effort would help the economy by removing the drag of enormous debt from people who would otherwise not be able to buy a home or pursue a more economically sound future.
“We’re giving people a chance to make it,” Mr. Biden said. “Not a guarantee. Just a chance to make it.”
Mr. Biden’s announcement was a presidential do-over. In the summer of 2022, he put in motion a plan to wipe out $400 billion in student debt for about 43 million borrowers. That was blocked by the Supreme Court , which said he exceeded his authority. In the months since, Mr. Biden has waived small amounts of debt using existing programs. But now he is attempting a larger effort closer to the scale of his first try.
The original plan relied on a law called the HEROES Act, which the administration argued allowed the government to waive student debt during a national emergency like the Covid pandemic. The justices disagreed after Republican attorneys general and others challenged the debt waiver plan.
The new approach is different.
For months, Mr. Biden’s Education Department has been developing regulations using a long process authorized by the Higher Education Act. Instead of an across-the-board waiver of debt, the new approach targets five groups of borrowers: those whose loans have ballooned because of interest; borrowers who have been paying for decades; those who have economic hardship; people who qualify for existing debt relief programs but have not applied; and people whose loans come from schools that have since been denied certification or have lost eligibility for federal student aid programs.
Administration officials said because the new approach is based on a different law, it is more likely to survive the expected challenges. They said lawyers for the White House and the Education Department have studied the Supreme Court ruling and have designed the new program to make sure it does not violate the principles laid out by the justices.
But lawyers for those who oppose the approach are likely to argue that waiving the debt is unfair to those who already paid back their loans or never took out college loans in the first place. That argument helped sway the justices in the last case.
Neal McCluskey, the director of the Center for Educational Freedom at the Cato Institute, called the new plan “dangerous policy” that is unfair to taxpayers and would cause colleges and universities to raise their prices.
“The Constitution gives Congress, not the president, the authority to enact law, and the Supreme Court has already struck down a unilateral, mass student debt cancellation scheme by the Biden administration,” he said. “It would stick taxpayers with bills for debts other people chose for their own financial advancement.”
The legal challenges will likely take months to resolve, and that could leave the debt relief plan in limbo as voters go to the polls in November to choose between Mr. Biden and former President Donald J. Trump.
Members of Mr. Biden’s administration fanned out across the country on Monday to talk about the new plan, betting that it will rally support among voters who were disappointed that the court blocked the first one, which would have eliminated up to $20,000 in debt for tens of millions of borrowers. Vice President Kamala Harris held a round-table discussion with a teacher, a nurse and a social worker in Philadelphia. Miguel A. Cardona, the education secretary, spoke in New York City.
“We need you to stay in these jobs doing this work,” Ms. Harris said in the library of an elementary school. “And you shouldn’t have to make a decision about whether you serve or are able to pay your bills.”
But beyond the threat of legal action, the president faces steep obstacles just because of the calendar. The new plan has not yet been published in the Federal Register, which will kick off a required, monthslong public comment period before it can take effect. Officials said on Sunday only that they hoped some of the provisions would begin going into effect in “early fall” of this year.
Administration officials hope that the president’s supporters will give him credit for trying, even if many of the borrowers do not end up seeing any relief before they go to the ballot box. Andrew O’Neill, the legislative director for Indivisible, a liberal advocacy organization, praised Mr. Biden’s announcement.
“Progressives have led the fight for student debt cancellation, and Joe Biden has responded,” he said in a statement. “More than 30 million folks will now get relief from Biden’s programs. That’s a huge deal.”
White House officials have been scrambling for months to respond to the anger about student loans among the president’s base. In one poll released last month, more than 70 percent of young people said the issue of student loan forgiveness was “important” or “very important” to them as they make their decision in the 2024 election campaign.
Officials said the five groups of people targeted in the new plan will address most of the egregious issues that some borrowers have with their student loans.
People whose loans have grown beyond the amount they originally borrowed because of interest would have up to $20,000 of that interest wiped away, leaving them to repay only the amount they originally borrowed. People making less than $120,000 a year, or couples making less than $240,000, would qualify to have all of their interest forgiven.
Officials said that 23 million people would most likely have all of their interest-related balances waived from that provision.
About two million borrowers who already qualify to have their student loans waived under existing programs have not applied for relief. Under the new rules, the Education Department would be authorized to cancel the debt for those people without their having to apply.
People who took out federal student loans for undergraduate degrees and began repaying them more than 20 years ago would automatically have the debt canceled under the new plan. Graduate students who borrowed money and began repaying 25 years ago would have their debt canceled.
Officials said that about 2.5 million people would qualify under that rule.
People who borrowed money to attend colleges that have since lost their certification or their eligibility to participate in the federal student aid program would have their debt canceled. Officials did not say how many people that would affect. And people who are especially burdened with other expenses — such as high medical debt or child care — could apply to have their student loans forgiven.
Officials did not estimate how many people might qualify for what they called the “hardship” programs.
Nicholas Nehamas contributed reporting from Philadelphia.
Michael D. Shear is a White House correspondent for The New York Times, covering President Biden and his administration. He has reported on politics for more than 30 years. More about Michael D. Shear
Our Coverage of the 2024 Election
Presidential Race
Days after saying that abortion policies should be left to the states , Donald Trump criticized an Arizona court ruling for upholding an 1864 law that banned nearly all abortions and said that he would not sign a national ban if elected .
A closely watched measure of inflation remained stronger than expected in March , dealing a political blow to President Biden , who has been banking on cooling inflation to lift his re-election prospects.
Trump once again criticized Jews who back Democratic candidates , saying that “any Jewish person that votes for a Democrat or votes for Biden should have their head examined.”
Trump’s penchant for bending the truth has been well documented, but a close study of how he does so reveals a kind of technique to his dishonesty .
Primaries in three Mid-Atlantic House districts will test whether the battle cry of “save democracy” will be enough even for Democratic voters who have many other concerns.
In Arizona’s crucial Senate race, Ruben Gallego, who has long embraced his progressive background, is striking a moderate tone .
Allies of Trump are discussing ways to elevate third-party candidates in battleground states to divert votes away from Biden.
- Web Stories
- Life & Women
- Society & Culture
World Health Day 2024: Best Short Speech In English For Students
World Health Day is observed globally on April 7th each year to raise awareness about health issues and to advocate for prioritising health and wellness. Short speeches delivered on such occasions often aim to inspire students and others to adopt healthy habits, overcome challenges, and recognise the benefits of making wellness a priority in their lives.
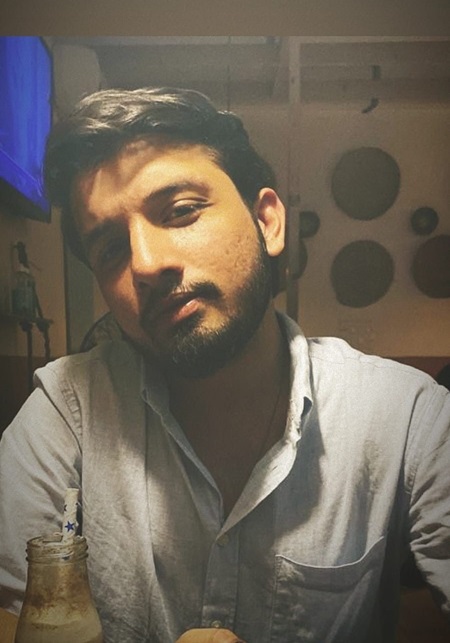
- Updated - 2024-04-06, 11:41 IST
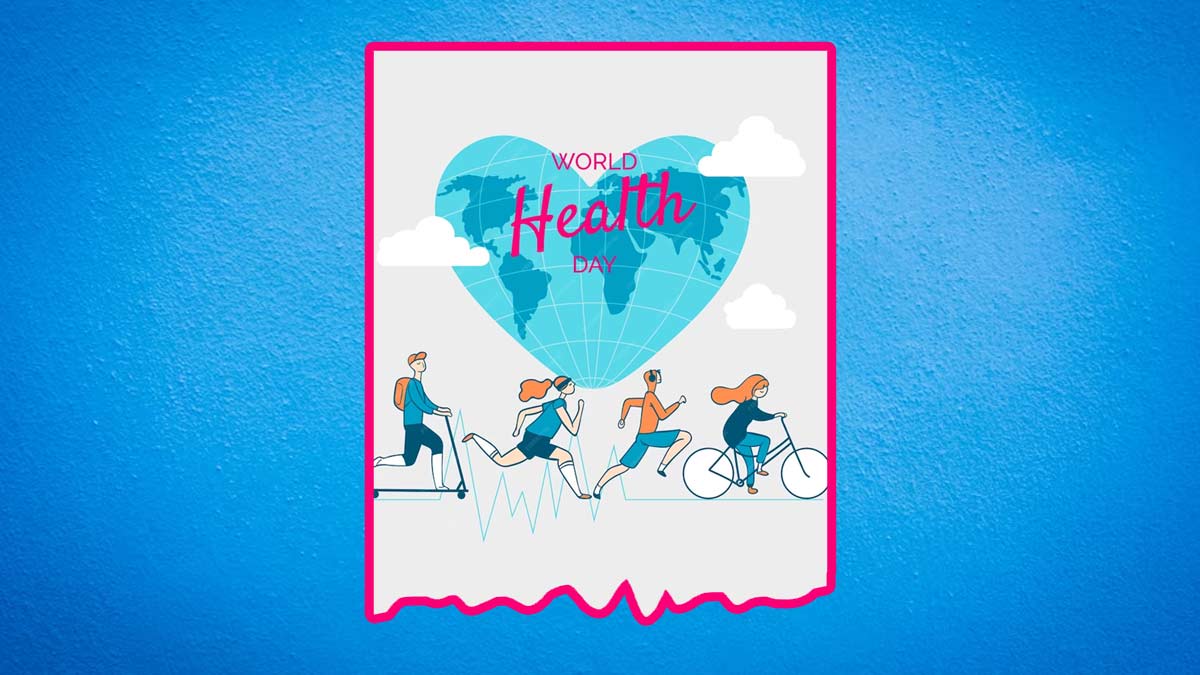
Speech 1: Investing In Your Most Valuable Asset
Speech 2: the power of prioritising your health.
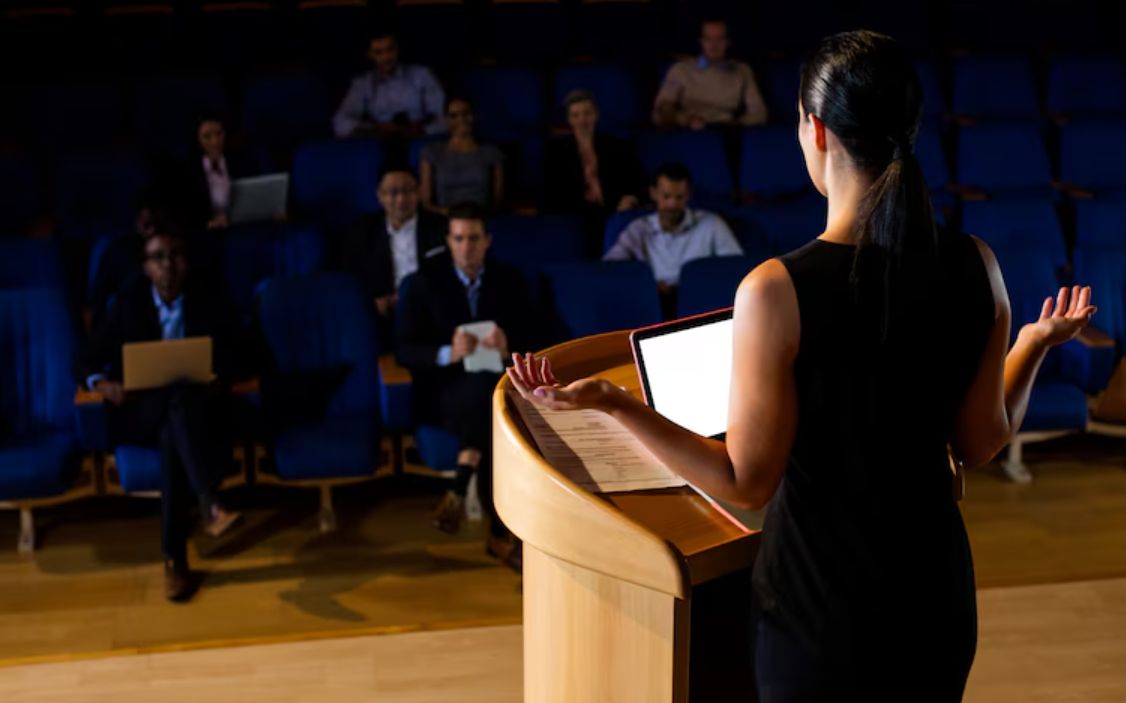
Speech 3: Your Health, Your Future
Image Courtesy: FreePik
Your skin and body like you are unique. While we have taken all measures to ensure that the information provided in this article and on our social media channels is credible and expert verified, we recommend you consult a doctor or your dermatologist before trying a home remedy, quick hack or exercise regime. For any feedback or complaint, reach out to us at [email protected]
- WEATHER ALERT Flood Warning Full Story
- student loans
Biden in Chicago for private campaign reception after speaking on student loans in Wisconsin
Protesters gathered on Michigan Avenue, as Biden held event at private residence near Drake Hotel

WASHINGTON -- President Joe Biden said Monday that college graduates would see "life-changing" relief from his new plan to ease debt burdens for more than 30 million borrowers, the latest attempt by the Democratic president to make good on a campaign promise that could buoy his standing with young voters.
Biden detailed the initiative, which has been in the works for months, during a trip to Wisconsin, one of a handful of battleground states that could decide the outcome of Biden's likely rematch with Donald Trump, the presumptive Republican nominee.
ABC7 Chicago is now streaming 24/7. Click here to watch
Biden said he wanted to "give everybody a fair shot" and the "freedom to chase their dreams" as he lamented the rising cost of higher education.
"Even when they work hard and pay their student loans, their debt increases and not diminishes," he said. "Too many people feel the strain and stress, wondering if they can get married, have their first child, start a family, because even if they get by, they still have this crushing, crushing debt."
Biden in Chicago
Biden was stopping in Chicago for a campaign event before returning to the White House late Monday.
He arrived at O'Hare airport after 2:30 p.m. before heading to the Soldier Field landing zone.
There, Rep. Danny Davis, Mayor Brandon Johnson and Cook County Board President Toni Preckwinkle greeted him.
The president's motorcade then headed to the Drake Hotel and a nearby residence.
A group of protesters demanding an end to Israel's attacks on Gaza gathered down the block Monday afternoon.
The private fundraising event includes only about two dozen people.
Biden's trip comes less than a week after primary voting in Wisconsin that highlighted political weaknesses for Biden as he prepares for the general election.
More than 48,000 Democratic voters chose "uninstructed" instead of Biden, more than double his narrow margin of victory in the state in 2020.
Trump also saw a significant number of defections during the state's primary, with nearly 119,000 Republicans voting for a different candidate than their party's presumptive nominee.
But Biden's results, which echoed similar protest votes in states like Michigan and Minnesota, have rattled Democrats who are eager to solidify the coalition that catapulted him into the White House in the first place.
A critical fracture has been the war in the Middle East. Young voters are more likely to disapprove of Biden's enduring support for Israel's military operation in Gaza, which has caused heavy casualties among Palestinian civilians.
Some have also been impatient with Biden's attempts to wipe away student loan debt. The U.S. Supreme Court last year foiled his first attempt to forgive hundreds of billions of dollars in loans, a decision that Biden called a "mistake."
Since then, the White House has pursued debt relief through other targeted initiatives, including those for public service workers and low-income borrowers. Administration officials said they have canceled $144 billion in student loans for almost 4 million Americans.
At the same time, the Department of Education has been working on a more expansive plan to replace Biden's original effort. Although the new federal rule has not yet been issued, Monday's announcement was an opportunity to energize young voters whose support Biden will need to defeat Trump in November.
Republicans said Biden's plan shifts the financial burden of college tuition onto taxpayers who didn't take out loans to attend school.
"This is an unfair ploy to buy votes before an election and does absolutely nothing to address the high cost of education that puts young people right back into debt," said Sen. Bill Cassidy of Louisiana, who chairs a committee on education and other issues.
Biden will make the announcement on Monday in Madison, the state's liberal capital and home of the University of Wisconsin's flagship campus. The president is scheduled to speak at a nearby technical college.
Nearly 15% of Democrats in Dane County, home to Madison, voted "uninstructed." That is nearly double the statewide total of 8%.
Democratic U.S. Rep. Mark Pocan, who represents Madison, said he was struck that concerns about Israel's war against Hamas in Gaza were top of mind among voters at five town halls over the past two weeks in more rural parts of his district.
"I was surprised to see the intensity on the issue of Gaza coming not from a student voice out of Madison, but older voters in more rural parts of the district," Pocan said.
Pocan said the number of "uninstructed" votes shows the concern in Wisconsin and that Biden needs to address it. He said he planned to talk directly with Biden about it on Monday.
"I just want to make sure he knows that if we're going to have a problem, that could be the problem in Wisconsin," Pocan said.
Biden's new debt plan would expand federal student loan relief to new categories of borrowers through the Higher Education Act, which administration officials believe puts it on a stronger legal footing than the sweeping proposal that was killed by a 6-3 court majority last year.
The plan is expected to be smaller and more targeted than Biden's original plan, which would have canceled up to $20,000 in loans for more than 40 million borrowers. The new plan would cancel some or all federal student loans for more than 30 million Americans, the White House said. The Education Department plans to issue a formal proposal in the coming months, with plans to start implementing parts of the plan as early as this fall.
"President Biden will use every tool available to cancel student loan debt for as many borrowers as possible, no matter how many times Republican elected officials try to stand in his way," White House press secretary Karine Jean-Pierre said in a call with reporters.
Details the White House released on Monday largely mirror a plan drafted by the Education Department. It lays out five categories of borrowers who would be eligible to get at least some of their federal student loans canceled if the rule is approved.
The plan's widest-reaching benefit would cancel up to $20,000 in interest for borrowers who have seen their balance grow beyond its original amount because of unpaid interest. Borrowers could get the entirety of their interest erased, with no limit, if they are enrolled in an income-driven repayment plan and have annual incomes of less than $120,000 or couples making less than $240,000.
That part of the plan would forgive at least some unpaid interest for an estimated 25 million borrowers, with 23 million getting all their interest erased, according to the White House.
An additional 2 million borrowers would automatically have their loans canceled because they're eligible but have not applied for other forgiveness programs, such as Public Service Loan Forgiveness.
Borrowers who have been repaying their undergraduate student loans for at least 20 years would be eligible to have any remaining debt canceled, along with those repaying their graduate school loans for 25 years or more.
The plan would forgive debt for those who were in college programs deemed to have "low financial value." It's meant to help those who were in programs that ended up losing eligibility to receive federal student aid or programs found to have cheated students.
A final category would cancel debt for borrowers facing hardships that prevent them from repaying their student loans, either because they're at high risk of defaulting or are burdened with medical debt or child care expenses, among other criteria.
Cardona said in a call with reporters that the relief that would be provided under the new plan would be "on top of the $146 billion in student loan debt relief for 4 million Americans that we've already approved, more than any other administration in our country's history."
Hearings to craft the rule wrapped up in February, and the draft is under review. The Education Department will issue a formal proposal and open it to public comment before it can be finalized.
ABC7 Chicago and Associated Press writer Scott Bauer in Madison, Wisconsin, and Chris Megerian in Washington contributed to this report.
Related Topics
- STUDENT LOANS
Student Loans
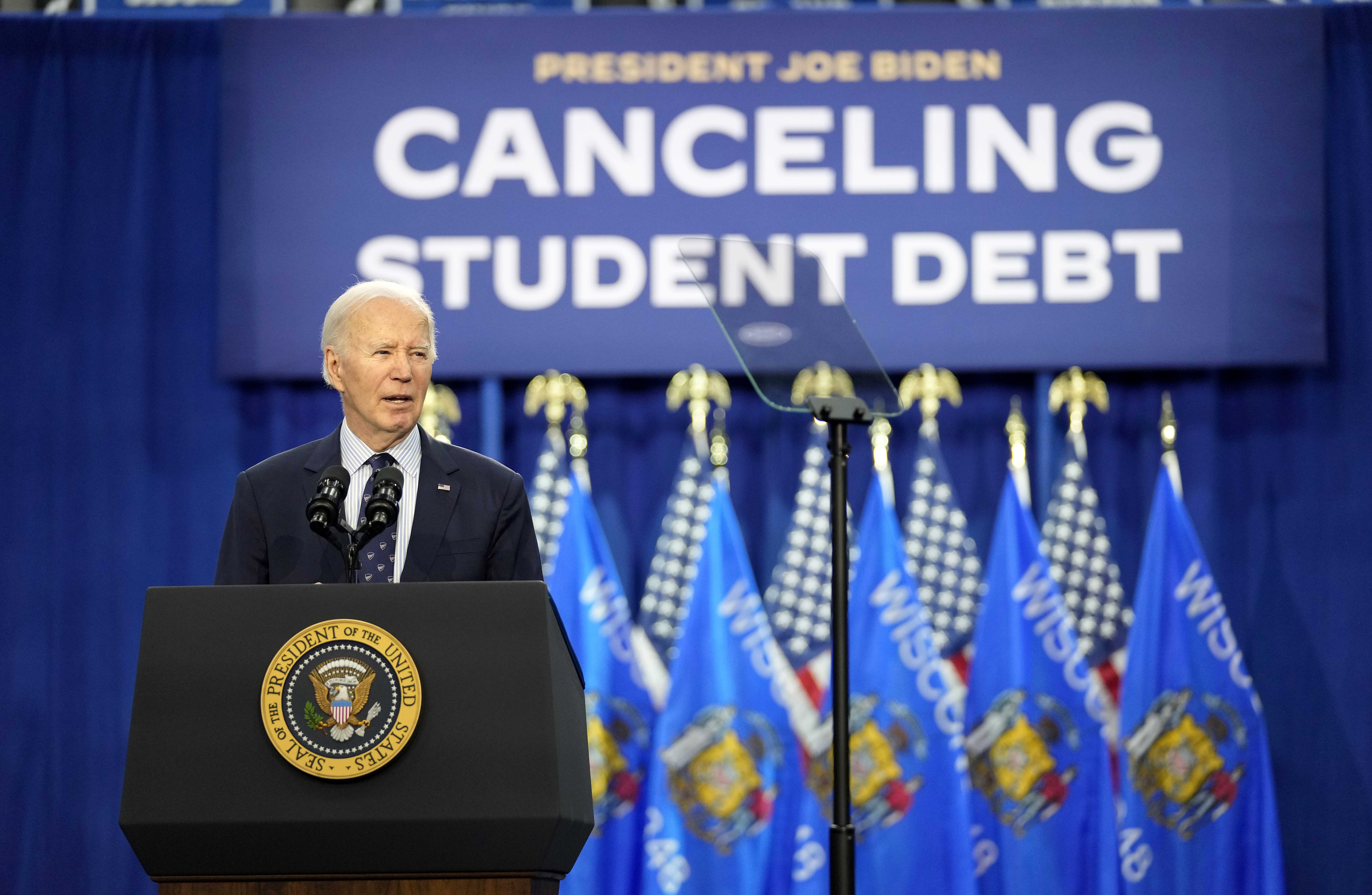
What to know about Biden's latest attempt at student loan cancellation
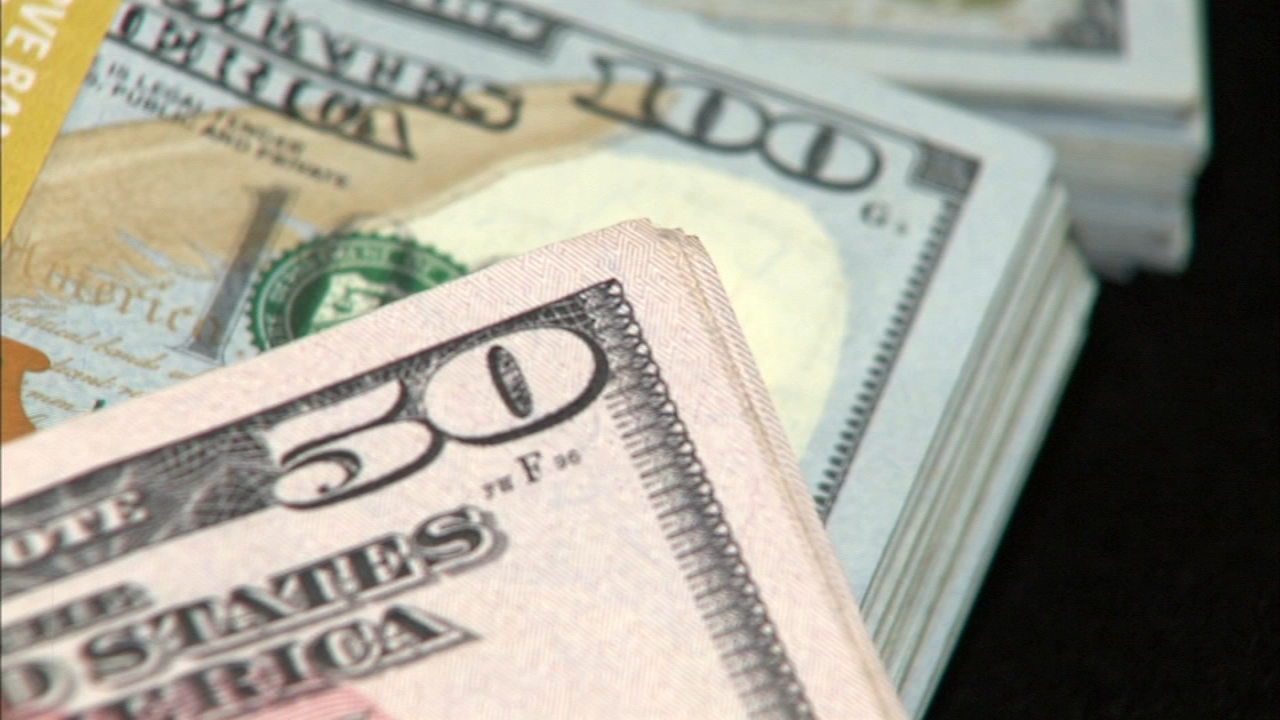
How you can lower your student loan payment, or get it forgiven
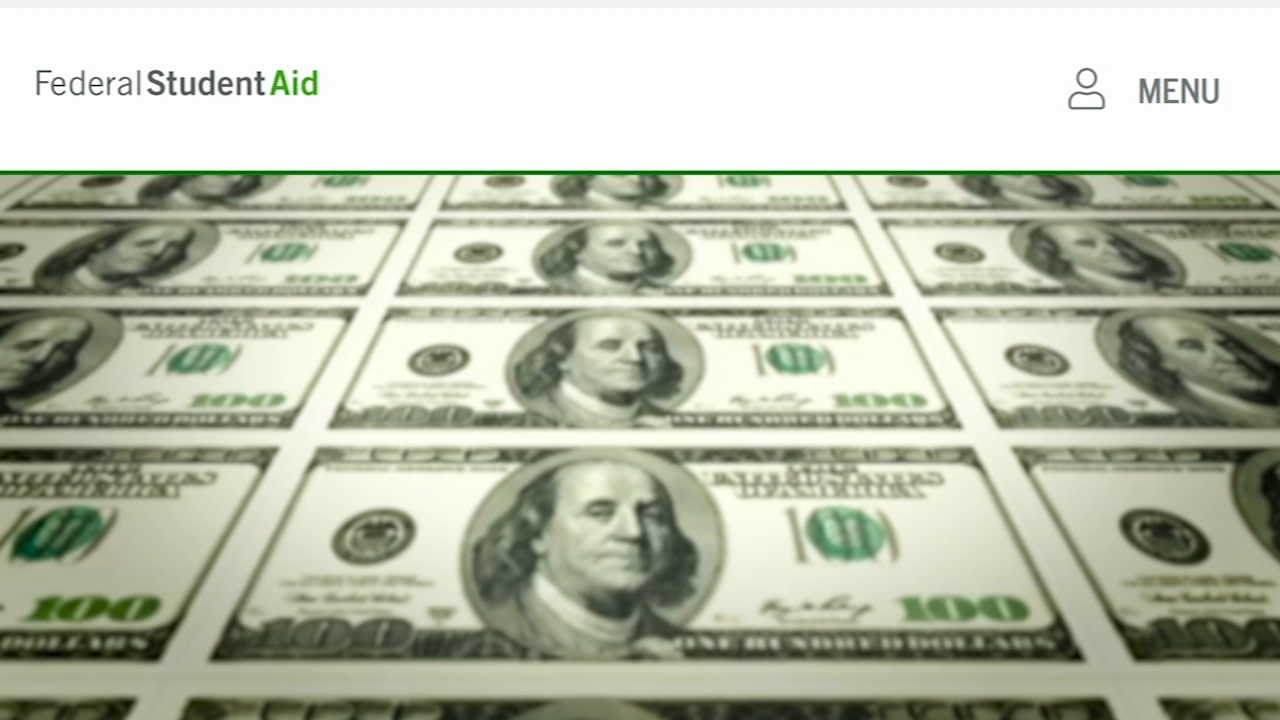
Public servants getting $5.8B in student loan forgiveness this week
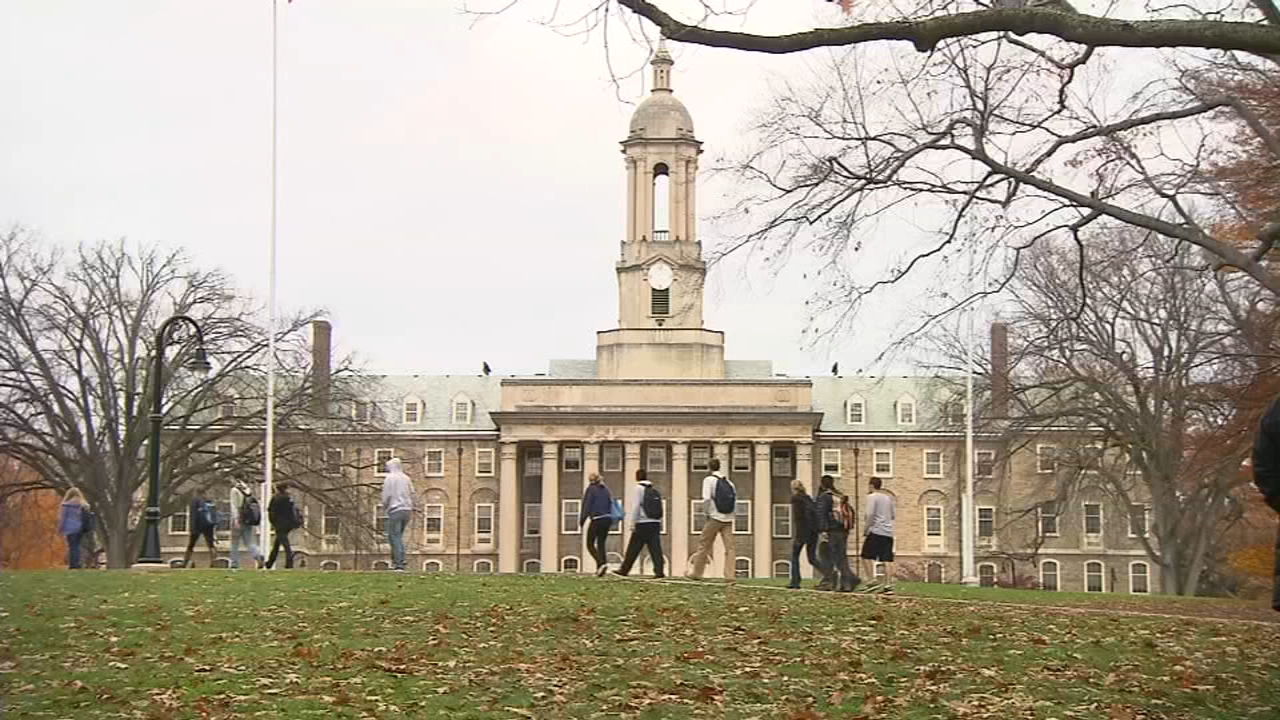
78K public service workers to have student loans forgiven
Top stories.
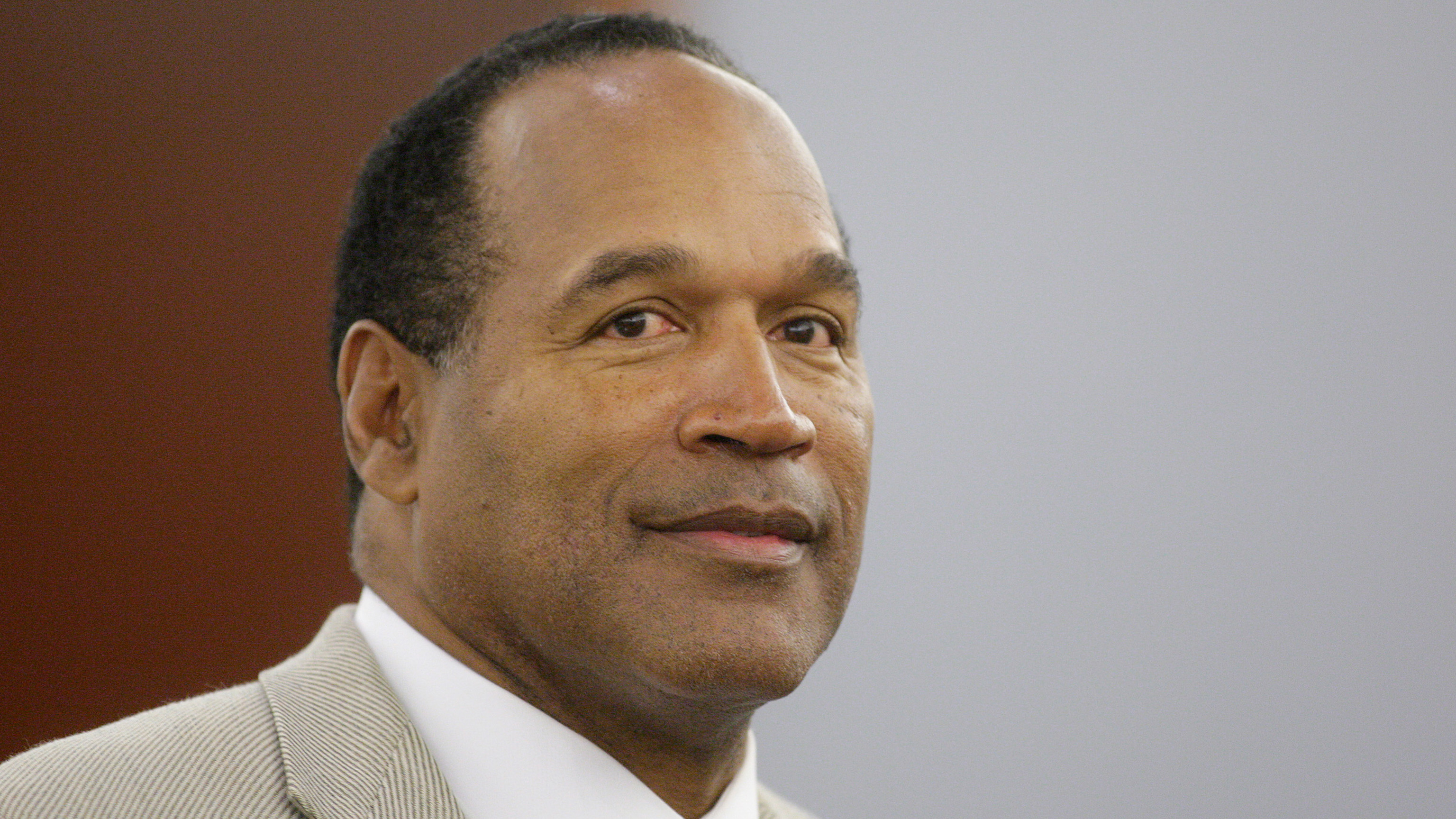
O.J. Simpson dies after battle with cancer, family says. He was 76
- 2 minutes ago
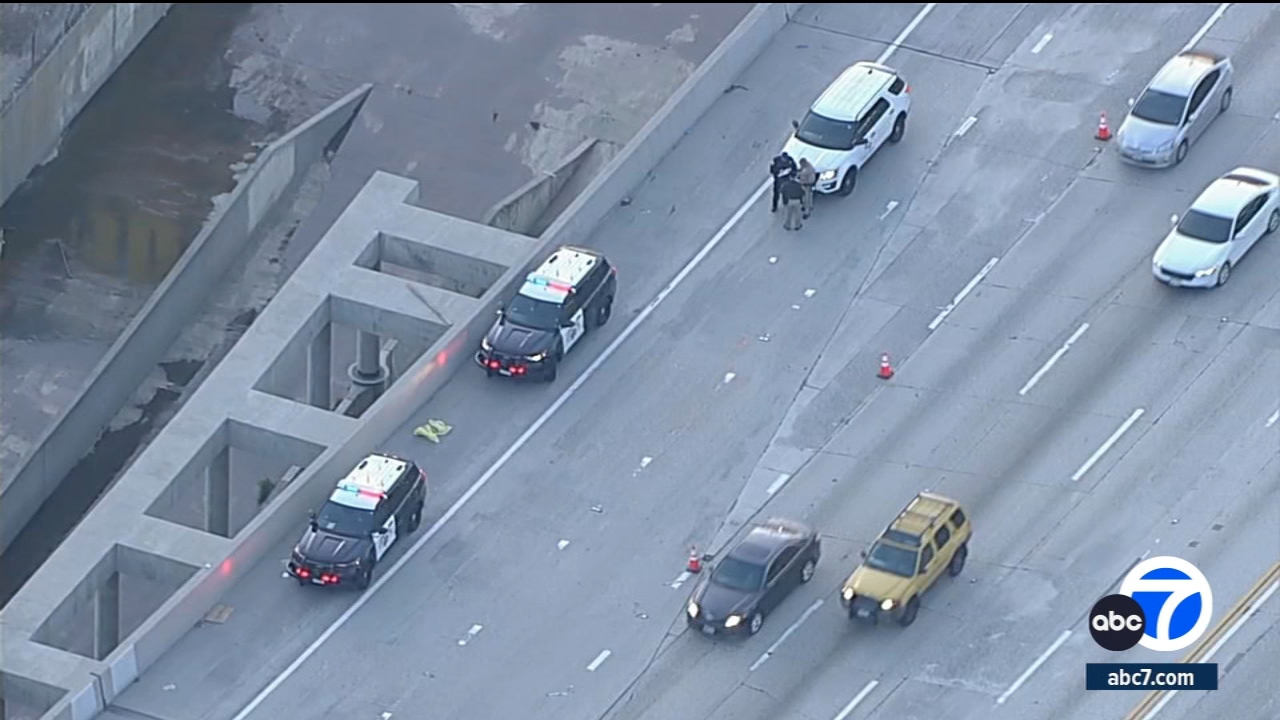
Woman posted about eclipse, apocalypse before pushing kids on freeway
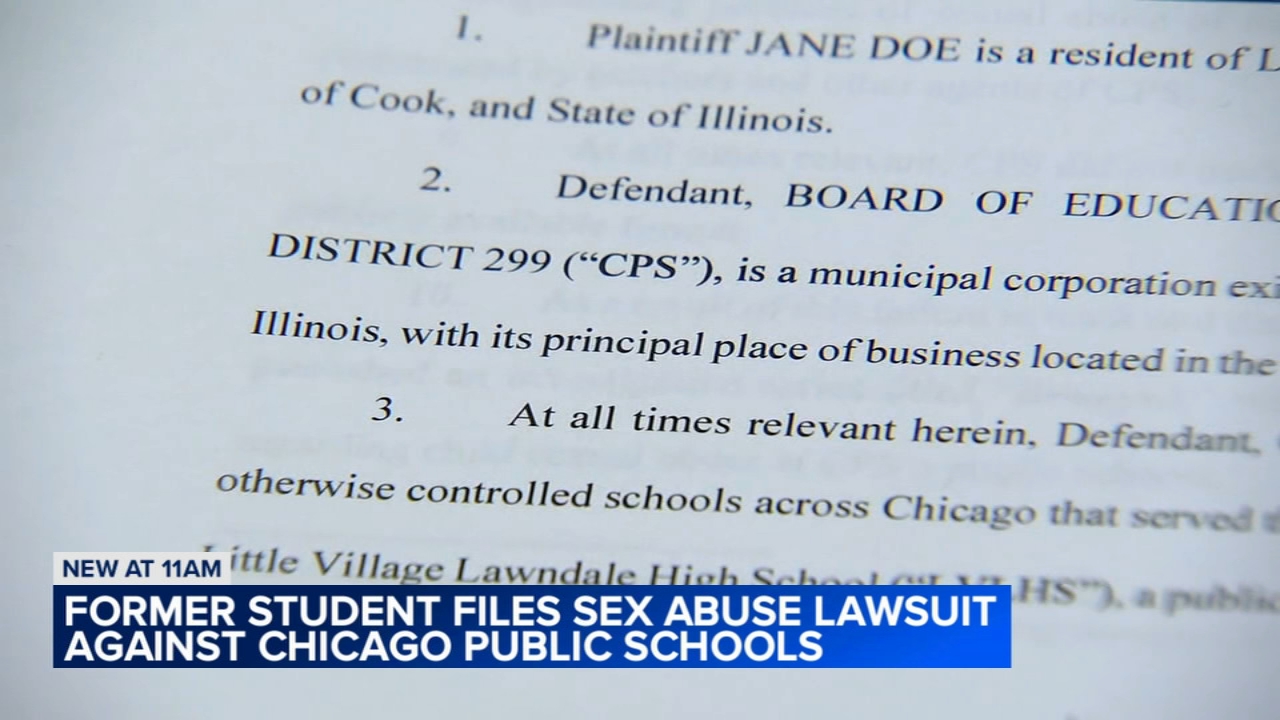
Former student files sexual abuse lawsuit against CPS
- 14 minutes ago
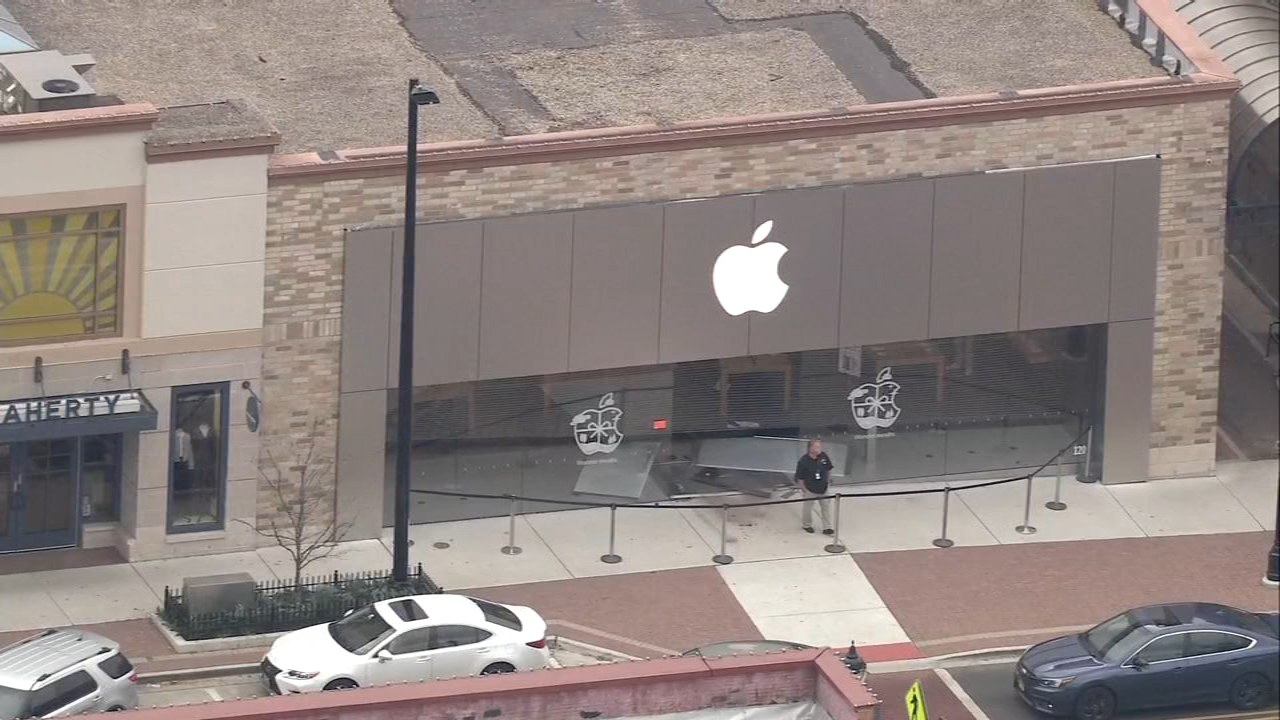
3 charged in crash-and-grab burglary at Naperville Apple Store: police
- 27 minutes ago
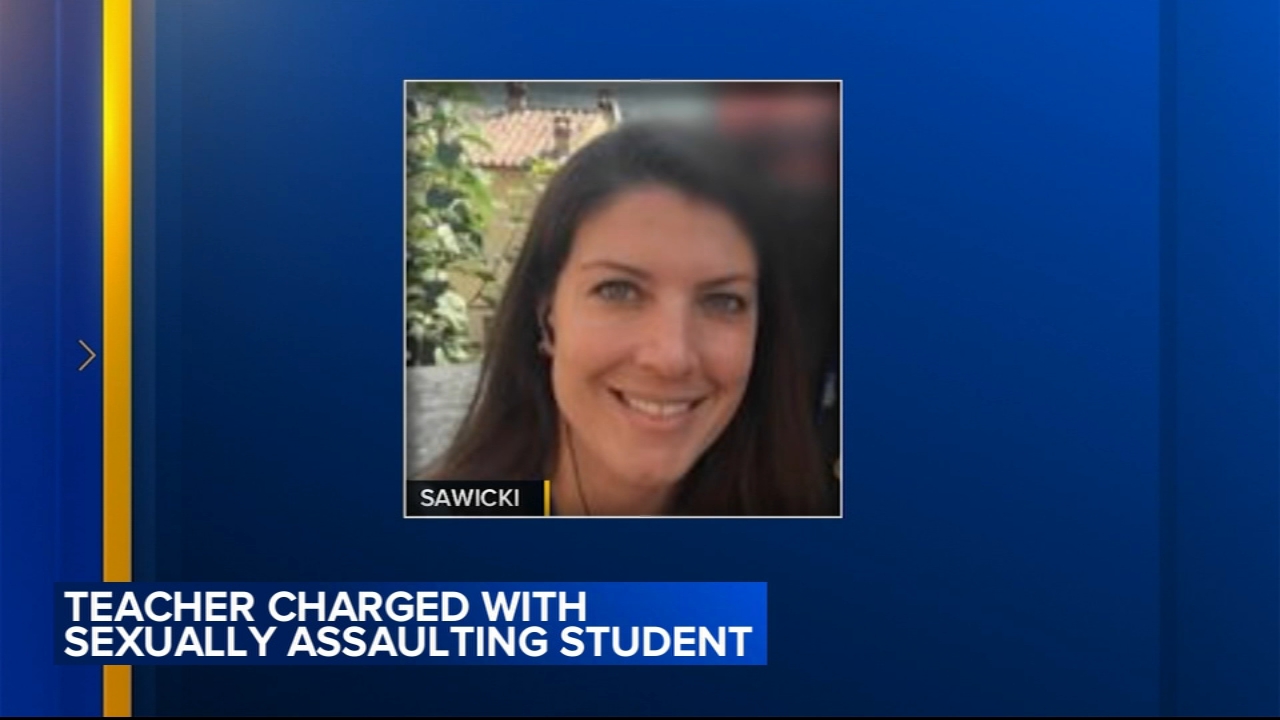
NJ teacher accused of sexually assaulting her student
- 2 hours ago
Students playing 'senior assassins' game mistaken for real gunmen
CPD tactical teams again under scrutiny after Dexter Reed shooting
CPD officers honored for investigating Auburn Gresham homicide
- 9 minutes ago
- Police, court and fires
- Urgent information
- Local sports
- Letters to the editor
- Engagements
- Anniversaries
- Welcome to Our World
- In The Schools
- Younger set
- Classifieds
- Garage Sales
- Submit News
- Terms of Service
- Browse Notices
- Place Notice

- Today's Paper
Subscribe Today
Rotary holds 4-way test speech contest.
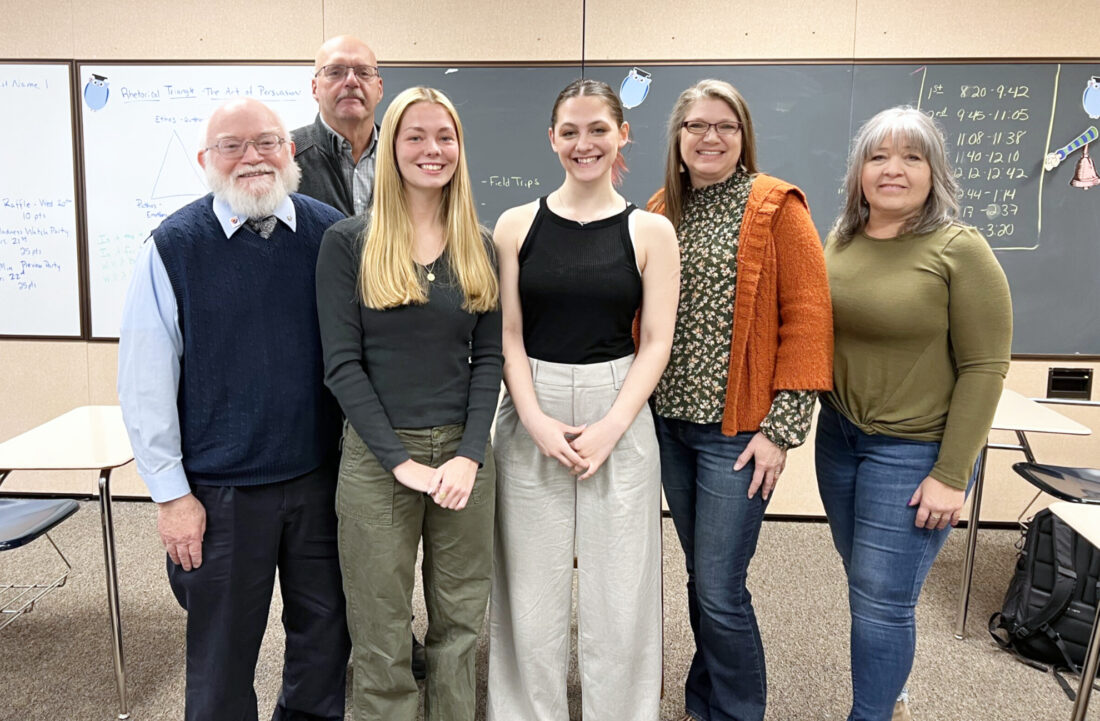
PHOTO PROVIDED Pictured are Lock Haven Rotary President Brent Jones (judge); Dr. Mark Rowedder (judge); Morgan Temple (runner-up); Ana Persun (winner); Rotarian Alison Kramer (organizer); and Nancy Hackenberg (judge).
The Rotary Club of Lock Haven held its annual 4-Way Test speech contest at CMHS on Tuesday, March 26. Students were required to write a 5-7 minute speech about an ethical issue and apply the tenets of the 4-Way Test:
1. Is it the truth?
2. Is it fair to all concerned?
3. Will it build goodwill and better friendships?
4. Will it be beneficial to all concerned?
The winner was Ana Persun and the runner-up was Morgan Temple.
Ana received a $100 cash prize and Morgan received $50. Ana moves on to the Regional Contest in State College on April 27. The winner from regionals moves on to the district finals on May 18 in Hagerstown, Md.
Many thanks go to Jenn Temple, CMHS teacher, for her time in working with the students to prepare for the contest.
Today's breaking news and more in your inbox
- Daily Newsletter
- Breaking News
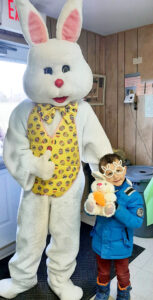
SV Lions and Easter Bunny visit
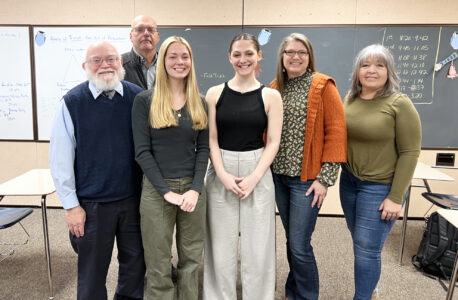
PennDOT manager offers glimpse of upcoming work projects
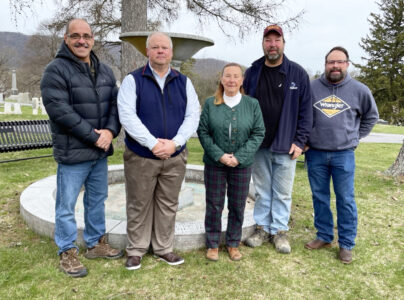
Bellefonte Elks and Union Cemetery
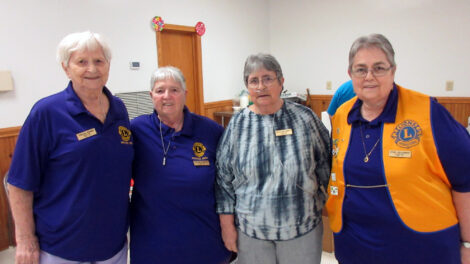
Howard Area Lions Club welcomes new members
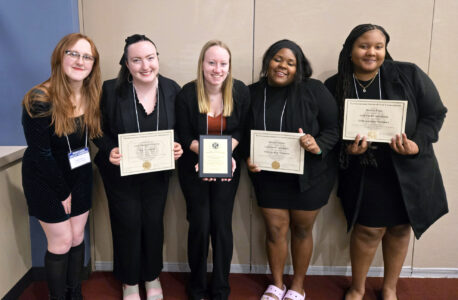
LHU Circle K Club wins awards
Starting at $3.69/week..

Conway creates public art ordinance after free speech debate over doughnut mural

A New Hampshire town's new ordinance that was pitched as "a path forward" for public artwork hasn't resolved a bakery owner's First Amendment dispute over a large pastry painting, and his lawyer predicts it will only lead to more litigation as town officials become "speech police."
Conway residents passed the ordinance by a vote of 1,277 to 423 during town elections Tuesday, part of a lengthy ballot for budget and spending items and picking government positions, such as selectboard, treasurer, and police commissioner.
The vote came more than a year after the owner of Leavitt's Country Bakery sued the town over a painting by high school students that's displayed across his storefront, showing the sun shining over a mountain range made of sprinkle-covered chocolate and strawberry doughnuts, a blueberry muffin, a cinnamon roll and other pastries.

Top stories of the day, 3X a week - subscribe today!
The zoning board decided that the painting was not so much art as advertising, and so could not remain as is because of its size. At about 90 square feet (8.6 square meters), it's four times bigger than the town's sign code allows.
The new ordinance requires applicants to meet criteria for art on public and commercial property. It says that while the zoning and planning boards must approve the appropriateness of theme, location, and design before the selectboard considers each proposal, the process should make "no intrusion into the artistic expression or the content of work."
"There's no part of writing that where we try to limit any kind of speech," Planning Board Chairperson Benjamin Colbath said at a March 28 meeting. "We did try to carefully write that and certainly took inspiration from what a lot of other communities are doing as well, as well as confirm with counsel on that one."
Top NHPR stories:
- Former head of White Mountain trail nonprofit accused of financial fraud
- 'No rats': Reporting YDC abuse led to retaliation, former staffers tell jurors
A lawyer for the bakery had urged voters to reject the ordinance.
"Typically, people get to decide whether to speak or not; they don't have to ask the government 'pretty please' first," Robert Frommer wrote last week in the Conway Daily Sun.
"All commercial property owners would have to get permission before putting up any sort of public art in town," Frommer wrote, and town officials can "deny murals because of what they depict, or who put them up."
Sean Young, the bakery owner, said he was voting NO: "Local officials don't get to play art critic."
Young sued after town officials told him the painting could stay if it showed actual mountains — instead of pastries suggesting mountains — or if the building wasn't a bakery.
Young's lawsuit was paused last year as residents considered revising how the town defines signs, in a way that would have allowed the sign to stay up. But that measure was seen as too broad and complex, and it failed to pass.
The mural remains in place for now, as his case heads to trial this November.
Frommer told The Associated Press in an email that the town hasn't said whether the new ordinance will impact Leavitt's mural, "and if Sean wanted to paint a different mural with the high school students at any of his businesses, he would have to jump through the ordinance's unconstitutional hoops."
The town's attorney didn't immediately respond to an emailed request for comment on Wednesday.
When Colbath discussed the ordinance at last month's meeting, he painted it as a way to facilitate more public art in town.
"There was a hole in our ordinance and I wanted to try to make it clear and an easier path forward for community art," he said.

You make NHPR possible.
NHPR is nonprofit and independent. We rely on readers like you to support the local, national, and international coverage on this website. Your support makes this news available to everyone.
Give today. A monthly donation of $5 makes a real difference.

IMAGES
VIDEO
COMMENTS
Opening your speech in silence can help enhance your speech in two ways. First, it will give the audience some time to settle in, post which you can expect to grab their dedicated attention. And secondly, silence would give you some time to understand the room and calm your pre-stage anxiousness. 6.
Tell them (Body of your speech - the main ideas plus examples) Tell them what you told them (The ending) TEST before presenting. Read aloud several times to check the flow of material, the suitability of language and the timing. Return to top. A step by step guide for writing a great speech.
Consider using a mirror or recording yourself to observe your body language and gestures. For instance, if you're giving a motivational speech, practice your gestures and expressions to convey enthusiasm and confidence. 8. Consider nonverbal communication.
It lets the audience know that the speech is about to end. Like the introduction, the conclusion can be broken into two parts: the review and the final statement. A. Review: During the first part of the conclusion, the speaker restates the topic of the speech and each main point. B. Final Statement: The speech ends with a strong final statement.
5. Deliver your speech to a mirror until you can do it without reading. Start by reading your speech out loud, then try to look down at the page less and less and make eye contact with your reflection instead. Eventually, you should be able to make your speech while only referring to notes written on index cards.
2. Engaging Opening. Captivate your audience from the start. Use compelling quotes, interesting facts, thought-provoking questions, or short anecdotes related to your topic. This will pique their curiosity and make your speech more receptive. 3. State the Purpose. Clearly state the purpose of your speech.
For you as the speaker, it's much easier (and more powerful) to tell a story that you lived versus one you read in a book. 2. Write out your speech from beginning to end. As Grant Baldwin discusses in this video on preparing your talk, you want to write out your talk to have a basic structure: beginning, middle, and end.
Speech length: 15 minutes with extra time for a 'Question and Answer' session at the end of the presentation. Speech title: How to win a future for your family when the kids need feeding, and the bills want paying. Introduction (2.5 minutes): Thanks for coming today … Summer heat, we'd all rather be at beach reading a book under a sun ...
Visit the room: If you have access to the classroom where you will be speaking outside of class hours, take the time to visit in advance and get used to standing at the front of the room.Make arrangements for any audio-visual equipment and practice standing in the exact spot where you will deliver your speech. Rack up experience: Volunteer to speak in front of your class as often as possible.
You need to write a speech in a way that keeps the attention of an audience and helps paint a mental image at the same time. This means that your speech should contain some color, drama, or humor. It should have "flair.". Make your speech memorable by using attention-grabbing anecdotes and examples.
Analyze their response and tweak the joke accordingly if necessary. Starting your speech with humour means your setting the tone of your speech. It would make sense to have a few more jokes sprinkled around the rest of the speech as well as the audience might be expecting the same from you. 4. Mohammed Qahtani.
Here are a few ways for you to do so: Tell a brief story. It should illustrate the main points of your speech. Summarize key points. This is to remind your audience of the purpose of your speech. There might have been points that your listeners zoned out on, so it's good to give them a brief run through of what was covered.
Writing the first draft should consume about 20% of your time as a speech writer. Develop a "hook.". You need to capture the audience's attention at the beginning of the speech and motivate them to keep listening. A humorous story or a startling statistic may serve this purpose, depending on the type of speech you're writing.
The opening. Start with an opening that hooks your audience before making the overall topic of your speech clear. Get their attention and prepare them to focus on the words that will follow. For ...
Timing and word count. Student Council Speeches are generally brief: around 1-4 minutes long which isn't a lot of time! That's between approximately 150 - 600 words at an average speaking rate of 150 words per minute. To be safe say your speech out loud as if you were delivering it for real and time it.
Words in a 3-minute speech. An average speech of three minutes in length would have roughly 390 words at a regular speech rate of 130 words per minute (wpm). Daphne Gray-Grant, a speech and writing coach, discovered that the typical speaking tempo is 125 to 150 words per minute or 375 to 450 words for a three-minute speech.
When delivering a student council speech, there are a few key techniques that can help make it more effective. 1. A Clear Starting Point: Start your speech by introducing yourself and briefly explain why you are running for office. Make sure to be extremely clear with your audience so they understand where you stand.
Here is an amazing farewell speech sample for students to learn how to write an amazing speech that will captivate the audience. 2013 Student Graduation Speech. Good morning family, friends, faculty, and fellow graduates. ... Timing: Respect the allocated time and write the speech accordingly. An overly long or short speech can diminish the ...
To write a student council speech, start with an attention-grabbing statement such as a question or a powerful quote about leadership. Next, briefly explain who you are, what position you are running for, and why you are running. Then list any relevant qualifications, such as a summer job. In the body of the speech, discuss at least 3 ways to ...
Demonstration speech topics and methods to develop hundred demonstratives for good public speaking, step by step. Follow those steps and read the tips. The goals could be numerous, i.e. to demonstrate a process and give the audience information while using visual aids, or to show how to do something, or how something works.. Begin your writing process by selecting some demonstration materials.
When given a topic to speak on, the first thing you can do is brainstorm ideas and pen down all that comes to your mind. This will help you understand what aspect of the topic you want to focus on. With that in mind, you can start drafting your speech. An opening statement can be anything that is relevant to the topic.
1. Choose a Topic For Your Debate. Also called a resolution or a motion, the topic is sometimes chosen to debate. This is usually the case in a school activity to practice debating skills. The resolution or motion is usually centered around a true or false statement or a proposal to change the current situation.
How to draw a picture. How to paint the best picture. How to tidy your room. How to tidy your desk. How to give a pet a bath. These are great how to presentation topics that can enable second-grade children to express their opinions with simple words and improve their writing skills.
A "how-to" speech template is an effective method to prepare a speech. This is especially useful when speaking on topics that may be largely unfamiliar to the audience, as it provides a straightforward structure for organizing the material. The basic format of this type of speech involves: Introducing the topic.
Mr. Biden's announcement was a presidential do-over. In the summer of 2022, he put in motion a plan to wipe out $400 billion in student debt for about 43 million borrowers. That was blocked by ...
Speech 1: Investing In Your Most Valuable Asset. Good morning, everyone. Today, on World Health Day 2024, I'm here to remind you all of the importance of prioritising your health. As students, we often find ourselves caught up in the whirlwind of academic responsibilities, extracurricular activities, and social commitments.
He arrived at O'Hare airport after 2:30 p.m. before heading to the Soldier Field landing zone. There, Rep. Danny Davis, Mayor Brandon Johnson and Cook County Board President Toni Preckwinkle ...
The Rotary Club of Lock Haven held its annual 4-Way Test speech contest at CMHS on Tuesday, March 26. Students were required to write a 5-7 minute speech about an ethical issue and apply the ...
The large painting of pastries created by students and displayed over the bakery is at the center of a legal battle pitting a zoning ordinance against freedom-of-speech rights. (AP Photo/Robert F ...